- 1Leptospirosis Research and Expertise Unit, Institut Pasteur de Nouvelle-Calédonie, Institut Pasteur International Network, Nouméa, New Caledonia
- 2Exact and Applied Sciences Institute (ISEA), University of New Caledonia, Nouméa, New Caledonia
- 3HydroSciences Montpellier, French Center for Scientific Research (CNRS), Institute of Research for Development (IRD), University of Montpellier, Nouméa, New Caledonia
- 4Institut de Minéralogie, de Physique des Matériaux et de Cosmochimie (IMPMC), UMR 7590 CNRS-MNHN-IRD, Paris, France
- 5Institut de Recherche pour le Développement (IRD), Nouméa, New Caledonia
- 6Institut Pasteur, Biology of Spirochetes Unit, French National Reference Centre for Leptospirosis, Paris, France
- 7Northern Province of New Caledonia, Koné, New Caledonia
- 8Pacific Community SPC – Public Health Division – B.P. D5, Nouméa, New Caledonia
Leptospira is a complex bacterial genus which biodiversity has long been overlooked. In the recent years however, environmental studies have contributed to shed light on its original and current environmental habitat. Although very fragile bacteria in laboratories, Leptospira have been shown to successfully occupy a range of soil and freshwater habitats. Recent work has strongly suggested that biofilm formation, a multicellular lifestyle regulated by the second messenger c-di-GMP, might be one strategy developed to overcome the multiple challenges of environmental survival. Within the genus, a minority of pathogenic species have developed the ability to infect mammals and be responsible for leptospirosis. However, most of them have retained their environmental survival capacity, which is required to fulfill their epidemiological cycle. Indeed, susceptible hosts, such as human, suffer from various symptoms, while reservoir hosts stay asymptomatic and release bacteria in the environment. In this review, we discuss how c-di-GMP might be a central regulator allowing pathogenic Leptospira to fulfill this complex life cycle. We conclude by identifying knowledge gaps and propose some hypotheses that should be researched to gain a holistic vision of Leptospira biology.
1. Introduction
Leptospirosis is an environment-borne bacterial zoonotic disease that is emerging or re-emerging in tropical and temperate regions. This disease is climate-sensitive and will likely further increase its burden in the coming decades as a consequence of climate change (Lau C. L. et al., 2010). While all mammals but mostly rodents can be reservoirs of pathogenic Leptospira and disperse virulent bacteria through their urine in soils and waters, humans are instead considered as incidental and susceptible hosts for the bacteria. The growing human population in peri-urban informal settlements or slums creates the ideal conditions for rodent proliferation resulting in an increased transmission of rodent-borne pathogens, including pathogenic leptospires (Costa et al., 2014). Although estimated numbers of infections support that leptospirosis is responsible for more than 1 million cases and almost 60,000 fatalities each year, corresponding to 1 new case every 30 s and one death every 9 min (Costa et al., 2015a), the disease still attracts insufficient research attention and funding (Goarant et al., 2019).
In recent years, there has been a renewed interest in unraveling the environmental component of leptospirosis epidemiological cycle, owing to the fact that human cases have largely been attributed to indirect infection from the environment (Bierque et al., 2020b). This has not only led to the discovery of an overlooked biodiversity of Leptospira spp. (Vincent et al., 2019), but also strongly suggested that soil is the original and major habitat of the Leptospira genus (Thibeaux et al., 2018). In the mammalian reservoir host, pathogenic leptospires live in the proximal renal tubules where they are hidden in an immune-privileged compartment of the body and are protected from environmental changes within the body of a homeothermic animal. Once leptospires are excreted through the urine of a reservoir mammal, they enter a highly variable environment with both biotic and abiotic features which challenge their survival. Within the kidney tubules, pathogenic leptospires are thought to live in a biofilm lifestyle (Santos et al., 2021). Bacterial biofilm is a particular and collective lifestyle, in which bacteria abandon a planktonic state, adhere to one another collectively and produce a protective extracellular matrix. This biofilm lifestyle was demonstrated in most Leptospira species in vitro (Ristow et al., 2008). More recently, biofilm formation was also shown to allow Leptospira to withstand simulated environmental stressors such as UV radiation, salinity, pH among others (Thibeaux et al., 2020). Hence, biofilm lifestyle was likely selected by Leptospira ancestors through evolution to cope with harsh environmental stresses. This ancestral inherited biofilm lifestyle is still observed in the current Leptospira genus (Ristow et al., 2008; Thibeaux et al., 2020). Biofilm formation is a complex process that, in Leptospira, seems to be dependent on a wide transcriptomic reprogramming (Iraola et al., 2016). Interestingly, the transition between the planktonic stage characterized by high motility and the biofilm or sessile lifestyle is also, at least partly, dependent on changes in the intracellular concentration of the second messenger cyclic dimeric guanosine monophosphate (c-di-GMP) (Thibeaux et al., 2020), positioning the latter as a key regulator that could control Leptospira's environmental life cycle (see Box 1).
Box 1. C-di-GMP and biofilm
Bacterial biofilms are multicellular aggregates embedded in a three-dimensional self-produced matrix that confers protection against adverse conditions such as desiccation, osmotic shock, and exposure to some toxic compounds, UV radiation and predators. The matrix formed by bacteria is held together by interconnected compounds, such as self-produced polysaccharides, proteins, extracellular DNA (exDNA), cell lysis products, and material from the surrounding environment. Specific chemical compounds of the matrix can be considered as biological markers of bacterial biofilms.
Biofilm formation commonly occurs in several steps. After attaching to a surface, bacteria proliferate and develop into microcolonies. They produce a protective matrix to form a mature biofilm from which some planktonic bacteria can escape to colonize another surface or possibly an animal host (Toyofuku et al., 2016).
Cyclic-di-GMP (c-di-GMP) is a highly conserved intracellular second messenger in bacteria (Jenal et al., 2017), critical for biofilm formation. More specifically, two antagonistic classes of enzymes, diguanylate cyclases (DGCs) and phosphodiesterases (PDE), regulate c-di-GMP production and degradation, respectively (Hengge, 2009). These enzymes are characterized by specific active catalytic domains that are highly conserved among bacteria; EAL or HD-GYP in PDEs and GGDEF in DGCs (Ryjenkov et al., 2005; Schmidt et al., 2005). Their activity allows a fine regulation of intracellular c-di-GMP levels in bacteria. For example, it has been shown that reduced c-di-GMP levels in Pseudomonas aeruginosa can prevent the initiation of biofilm development, whereas increased levels can promote biofilm formation (Hickman et al., 2005). It is now generally accepted that c-di-GMP intracellular concentration increases during the early stages of biofilm formation and peaks during the mature biofilm phase. Bacteria that are released in a planktonic form regain a low level of c-di-GMP, which favors their motility and their ability to colonize other niches or new hosts (Valentini and Filloux, 2016).
In this review, we revisit Leptospira epidemiological cycle, starting with the environment, from which most infections arise, and which therefore represents a form of reservoir that is important to better understand. We then explore the mechanisms at play during the infection of a host in this environment, including the factors that influence the risk of spreading the disease. We also review the literature that allows a better understanding of the establishment of chronic renal carriage in a host that is also qualified as a reservoir and may contaminate the environment. This complex zoonotic cycle is envisioned through the lens of the c-di-GMP-dependent regulation of Leptospira lifestyle, which would allow the bacteria to adjust to the much-needed changes between motility and protection during the multiple transition steps of this complex cycle. Overall, we not only review the current scientific knowledge, but also suggest and discuss some hypotheses and attempt to identify the major gaps that need to be researched to better comprehend this cycle and create novel control strategies.
2. Environmental maintenance and dissemination of Leptospira
Over the last 10 years, the Leptospira genus has considerably expanded, steeply rising to 69 validly described species in 2022 (Vincent et al., 2019; Fernandes et al., 2022). Among them, eight are considered as the most virulent and are frequently involved in severe leptospirosis in both human and animal (P1 high-virulence species, Figure 1A). Interestingly, these have seldomly been isolated from environmental sources. However, studies indicated their ability to persist in soils for an extended period of time (Smith and Self, 1955; Okazaki and Ringen, 1957; Hellstrom and Marshall, 1978; Thibeaux et al., 2017; Stone et al., 2022), with intact capability to induce infection (Bierque et al., 2020a). The remaining 61 Leptospira species were all found in environmental sources, and most were exclusively isolated from the environment, highlighting their propensity to thrive and persist in natural environments. This characteristic, however, could also be attributed to their fastidious growth requirements, which pose challenges in isolating them from animals (Figure 1A). Interestingly, although poorly studied and only on a small set of genes, the chronology of the emergence of the genus Leptospira (Kurilung et al., 2019) suggests a probable apparition of the first Leptospira ancestor on Earth around 2 billion years ago. By contrast, pathogenic Leptospira speciation has been estimated to have occurred 250 million years ago, which is concomitant with the dinosaurs' decline, the latter being progressively replaced by mammals (see Figure 1B). This is consistent with the reduced environmental survival observed for some pathogenic species, such as Leptospira borgpetersenii for instance, which seems to be correlated to their genome reduction and consequent partial loss of genes. Part of these genes could be involved in the “environmental lifestyle” of leptospires and their loss might have contributed to the bacteria's evolutionary shift towards a more parasitic lifestyle (Bulach et al., 2006). However, Hornsby et al. have recently shown that L. borgpetersenii serovar Hardjo can still be cultured and are able to chronically infect hamsters after a persistence period of 8 months in tap water. Their isolation method suggests that care should also be taken when choosing the medium for environmental recovery (Hornsby et al., 2020).
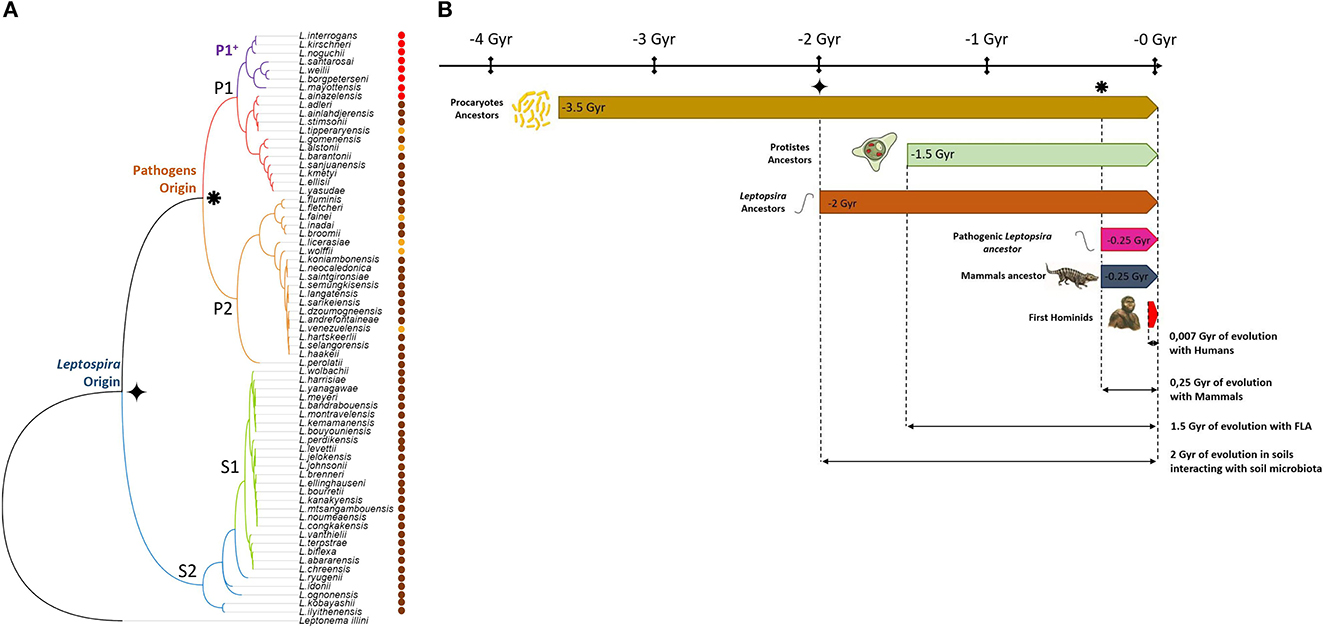
Figure 1. Environmental origin of Leptospira. (A) Phylogenetic tree based on 16S rRNA sequence of the 69 members of the Leptospira genus. Red dots denote highly virulent species isolated from animal samples. Brown dots show species exclusively isolated from environmental source, including low virulent species from P1 clade. Other species isolated from both animal and environmental sources are marked with an orange dot. (B) While first leptospires appeared 2 Gyr ago (), it is only 250 million years ago (
) that pathogenic strains arose. Information presented in this figure underscores the deep interconnections between leptospires and the environment throughout their evolutionary history. Over the course of 2 billion years, leptospires have developed mechanisms to persist and thrive in natural environments, particularly in soils. The presence of Leptospira species predominantly in environmental sources, along with their ability to persist in soils, suggests a strong ecological association. Furthermore, the coexistence of ancestral Leptospira with free-living amoebae in soils for over 1.5 billion years supports a longstanding mutualistic relationship between these organisms that share the same biotope. During this time, the environment, including amoebae, may have played a crucial role as host for Leptospira. These mutual dependence and adaptation to environmental conditions provide insights into the intertwined evolution of leptospires and their ecological niches. The relatively recent adaptation of certain pathogenic species to mammals further highlights the evolutionary trajectory of leptospires. Indeed, while the ancestral Leptospira were primarily adapted to environmental persistence, pathogenic strains have acquired the ability to infect mammalian hosts only recently, likely building upon their adaptations to soil environments.
While pathogenic species have specifically evolved to infect mammals over the last 250 million years, they still carry almost 2 billion years of environmental adaptation. This could explain why species that did not co-evolve to infect mammals have a full and intact ability to survive in the environment and are found so frequently across different climates worldwide. More than one century after its initial description, leptospirosis is increasingly recognized as an environment-borne infectious disease, in which not only water, but soil, plays a pivotal role in its cycle. How pathogenic leptospires manage to fulfill this complex cycle with multiple transitions remains largely unknown and deserves further studies.
2.1. Detection in soils
High numbers of isolation from soil and water have now re-emphasized the fact that the environment constitutes the original habitat of many Leptospira species (Scialfa et al., 2018; Bierque et al., 2020b; Miller et al., 2021; Yanagihara et al., 2022). Soils are considered as a central compartment in Leptospira lifecycle which supports environmental cycling and transmission, acting as an environmental reservoir or at least a temporary carrier of pathogenic strains. Investigating leptospirosis as an ecosystem-borne bacterial disease is now increasingly being considered, especially in a One Health perspective, which is a perfectly adapted approach to consider all aspects of this infectious disease (Sykes et al., 2022).
Several factors have been identified as affecting the persistence of Leptospira in soils. Leptospires were most frequently associated with soils of high organic matter content and moisture (Okazaki and Ringen, 1957; Henry and Johnson, 1978). For example, a Leptospira sp. from serogroup Pomona has been found to have a survival time of up to 193 days in a water-saturated soil, in contrast to only 5 days in a damp soil (Okazaki and Ringen, 1957). Furthermore, clay is known for its ability to absorb proteins (Schmidt and Martínez, 2016) and organic matter (Hong et al., 2019). These unique properties may play a critical role in promoting Leptospira soil colonization due to increased sorption capacity on the soil matrix (Smith et al., 1961), and could provide favorable conditions for their persistence (Islam et al., 2022). In addition, soil pH, salinity, temperature, and the presence of accompanying microorganisms are also critical parameters that influence the persistence of Leptospira (Parker and Walker, 2011). The presence of pathogenic leptospires has been positively correlated with soil nutrients such as nitrate, but also with metals such as iron, manganese and copper (Lall et al., 2018).
Viability qPCR on environmental DNA has successfully been used to demonstrate that pathogenic Leptospira can be found alive in soil and superficial sediment samples (Thibeaux et al., 2017). Successive culture isolation, five months apart, of identical species from the same soil has also been reported in Japan (Saito et al., 2013). Interestingly, this latter study suggested that the depth of isolation was related to the soil water content. Furthermore, Chiani et al. (2023) have recently shown that the hydrometeorological conditions, and therefore the water availability, were a critical parameter for Leptospira species detection in soils. This pattern is also suggested by some of our unpublished data. Taken together, we propose the hypothesis that leptospires may adjust their depth position in the soil to allow for a trade-off between the competing needs for water and oxygen, which gradients are frequently in opposite directions (Figure 2). Of note, further soil parameters such as moisture, nutrient availability, roughness, microbiota composition and hydroclimatic conditions may have an influence on Leptospira persistence (Chiani et al., 2023). Importantly, biofilm-forming Leptospira may also successfully persist in soil upper layers even under unfavorable environmental conditions thanks to their increased ability to resist a wide variety of stresses.
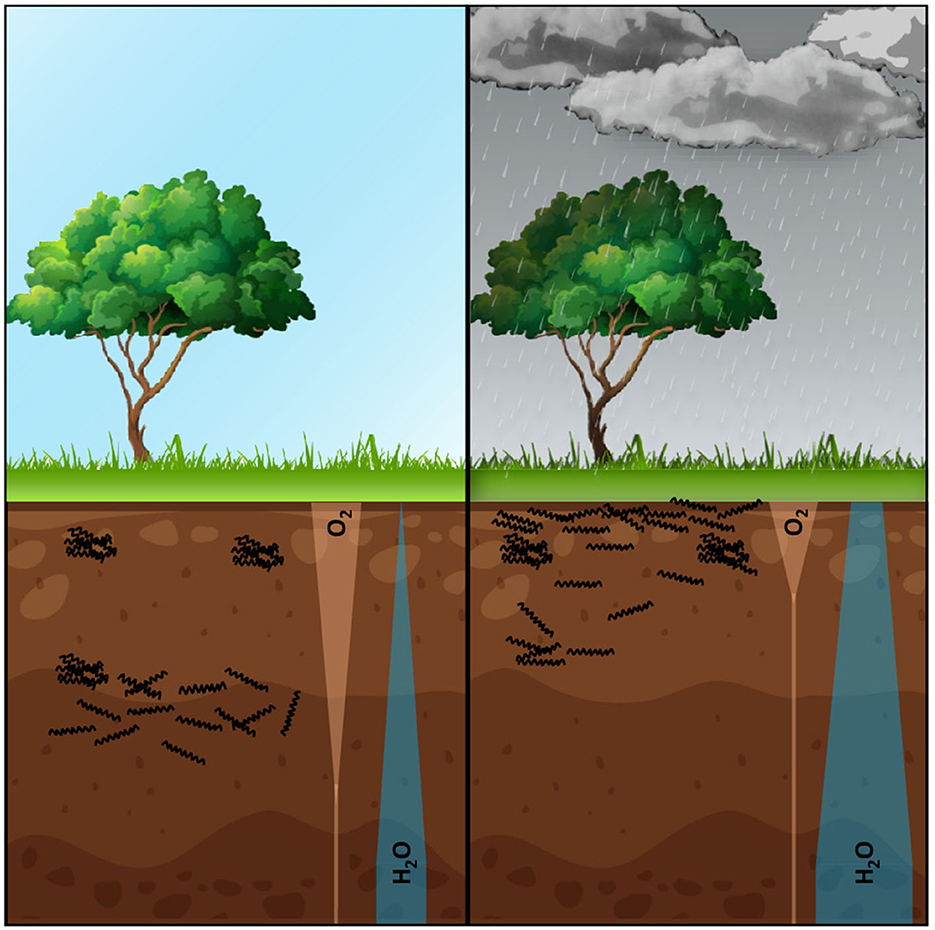
Figure 2. This figure illustrates our hypothetical scenario, in which leptospires face a trade-off between their need for oxygen and their need for water as they adapt to different depths within the soil. After rainfall, the oxygenated portion of the soil is significantly reduced and shifted towards the surface due to water saturation. In order to survive, aerobic leptospires would have to adjust their depth in the soil or endure these unfavorable conditions by forming a biofilm. By modulating their positioning within the soil, leptospires may migrate closer to the surface to take advantage of the increased oxygen availability, while still maintaining access to the necessary moisture for survival. This dynamic adaptation would enable leptospires to balance their respiratory needs with the water requirements essential for their persistence. However, if the water-containing zone is limited, forming a biofilm becomes a strategy that would allow leptospires to withstand potential fluctuations in water availability, as it is known that components of bacterial biofilm matrix are highly hydrophilic and may act as water retainers. Such biofilm not only confers physical protection but also facilitates the exchange of essential nutrients and metabolites within the microbial community. This hypothesis provides a potential explanation for the observed pattern of human contamination following floods. If the pathogenic Leptospira load in the environment, released by chronic carrier mammals, is substantial, floods can serve as a mechanism for dispersing bacteria and increasing the risk of human exposure. The disrupted soil structure and the release of Leptospira from the biofilm during flooding events may thus contribute to the widespread contamination observed in flood-affected areas. Note that this is a hypothetical scenario, and further research is required to challenge and refine this hypothesis. Future investigations examining the depth distribution of leptospires in soils, their response to changes in oxygen and water availability, and the role of biofilm formation could provide valuable insights into the adaptation strategies of leptospires in soil environments.
2.2. Soil colonization and survival
Microcosms have been designed to study Leptospira persistence and survival in environment-like conditions (Casanovas-Massana et al., 2018b). These soil microcosm designs have been used to confirm survival and virulence after 6 weeks in soils. More recently, similar approaches demonstrated the ability of pathogenic Leptospira to multiply in a waterlogged soil mimicking a flooding event (Yanagihara et al., 2022). In this study soils were autoclaved, precluding to consider microbiota contribution to this survival; nonetheless, this still indicates that soil resuspension is prone to promote proliferation of pathogenic species from the P1-highly-virulent sub-clade. These results lead to question soil quality, in terms of bacterial biodiversity or community composition, as an additional indicator to predict soils capacity to allow pathogenic Leptospira survival. Identifying the environmental bacteria associated with leptospires in soil and understanding the interaction network in relation to their ability to persist in this ecosystem is a major question that needs to be answered to fully understand the environmental colonization, survival, and persistence of leptospires.
Metabarcoding of environmental DNA has been used to address the presence of pathogenic Leptospira species and potential host organisms, as well as microbiomes associated with Leptospira persistence in the environment (Sato et al., 2019). In Japan, this approach has revealed that 15 environmental bacterial species were significantly correlated with Leptospira detection, and most of these potentially Leptospira-associated bacteria were Proteobacteria (Sato et al., 2019). Interestingly, one intracellular bacterium Chlamydiae was also significantly correlated with Leptospira detection. Of note, there is recent evidence for diverse chlamydial symbionts including Rhabdochlamydiae within free living amoeba (Haselkorn et al., 2021). We hypothesize that leptospires may likewise be able to survive phagocytosis by free-living amoeba, with which they have shared the environmental habitat for 1.5 million years (Figure 1). In vivo and in vitro data are now available regarding the micro- and macro-organisms associated or co-existing with Leptospira biofilms which may support its survival outside an animal host (Meganathan et al., 2022). For instance, Leptospira forms biofilms with Staphylococcus aureus in vitro (Vinod Kumar et al., 2019). Further, pathogenic leptospires were found associated with several bacterial species in environmental biofilms (Kumar et al., 2015). Interestingly, biofilms formed in vitro with both Leptospira and Azospirillum brasilense demonstrated high resistance to antibiotics and environmental stresses (Kumar et al., 2015). These multiple resistances would provide a reliable environmental selective advantage over bactericidal antibiotics naturally present in the environment, allowing successful niche colonization and persistence.
Although cycling and transmission of Leptospira species are now well-described, environmental factors that influence these parameters are not well-understood (Ganoza et al., 2006). As mentioned before, pathogenic Leptospira can form biofilms and cell aggregates in vitro (Ristow et al., 2008) and in vivo (Ackermann et al., 2021; Santos et al., 2021). Whether they also use this strategy to meet the multiple challenges of environmental survival is very likely (see Figure 3). Indeed, biofilm lifestyle has been shown to increase the survival to simulated environmental stressors (Thibeaux et al., 2020). Furthermore, pathogenic Leptospira have indeed been evidenced in environmental biofilms in natural settings (Vinod Kumar et al., 2016). Interestingly, Leptospira biofilm has also been shown to be poorly adherent and to maintain some form of motility (Thibeaux et al., 2020), which might be used in the environment to escape hostile conditions (see Figure 1). Adopting this strategy might also contribute to limit dilution in the environment and to maintain sufficient concentration to achieve infection of a new host.
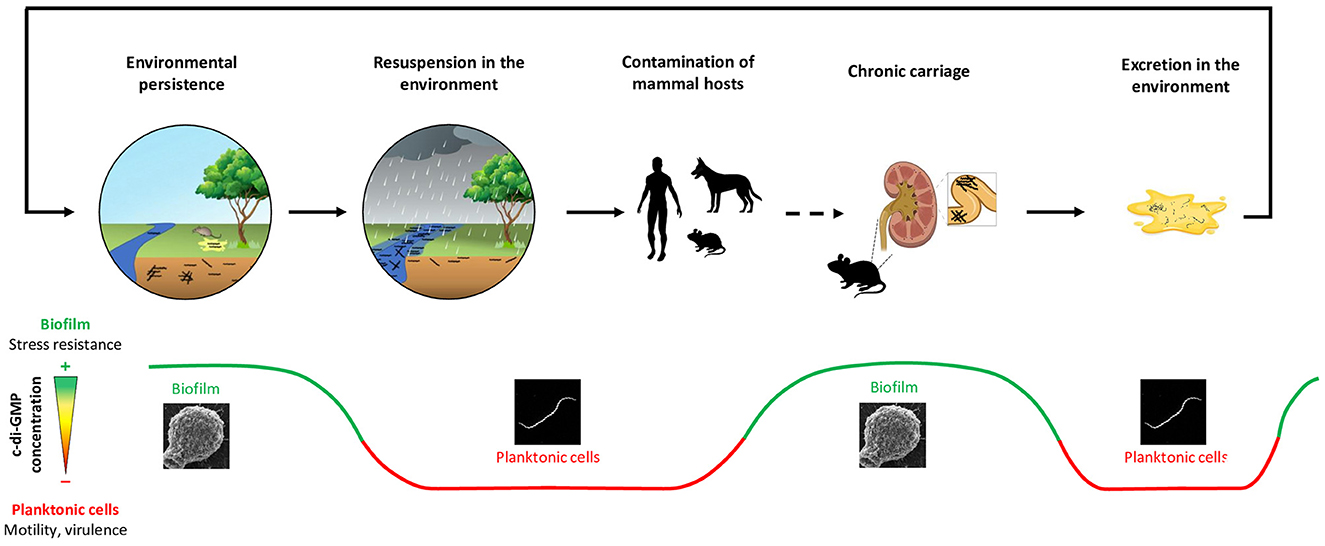
Figure 3. This figure illustrates a hypothetical model of c-di-GMP levels oscillations during the Leptospira epidemiological cycle, a process that is thought to play a crucial role in the survival and adaptation of these bacteria in diverse and challenging environments. The fine-tuned regulation of intracellular c-di-GMP levels may allow leptospires to navigate through different stages of their complex life cycle and overcome the transitions they encounter. Should Leptospira species adopt a biofilm strategy within soil habitats, heavy rainfall and consequent turbulence could potentially disrupt the biofilm, propelling leptospires into a planktonic stage. This transition may be associated with a decrease in the intracellular c-di-GMP concentration, which could have a twofold impact. On the one hand, the decreased c-di-GMP concentration would stimulate leptospires' motility, thereby facilitating their movement through water and their colonization of new habitats including hosts conducive to their survival. On the other hand, this decrease would stimulate the overexpression of virulence factors, amplifying the pathogenic potential of leptospires and enabling them to infect new mammalian hosts more effectively. Upon infection of a mammalian host, leptospires have the potential to establish a chronic colonization of the proximal kidney tubule. This crucial stage in the bacteria's lifecycle is thought to involve the formation of a biofilm, likely prompted by an increase in intracellular c-di-GMP levels. Finally, the process of urination presents an opportunity for pathogenic leptospires to detach from their biofilm state and be excreted in the environment. Upon detachment, these bacteria transition towards mobile planktonic cells, free to explore their surroundings and find new opportunities for growth or infection. These planktonic cells are then confronted with the need to adapt once again to their new environmental conditions. This adaptation may involve reverting back to a biofilm state, a process potentially facilitated by an increase in intracellular c-di-GMP concentrations. This cyclic adaptation process, from biofilm to planktonic cells and back again, underscore their ability to persist in diverse conditions, emphasizing the crucial role of c-di-GMP in the Leptospira epidemiological cycle. While this scenario remains theoretical and currently lacks direct empirical support, it provides a credible explanation for observed correlations between rainfall patterns and leptospirosis outbreaks, as well as the biological mechanisms that underpin leptospiral survival and transmission. Future investigations are essential to corroborate this hypothesis and elucidate the precise mechanisms by which c-di-GMP levels are modulated throughout the Leptospira epidemiological cycle.
Further studies are needed to unravel the lifestyle of Leptospira in the environment. Our hypotheses, together with those proposed by Vasconcelos et al. (2023), suggest that the regulation of c-di-GMP and the biofilm formation should be taken into account in future studies, as they may be key to bacteria survival and persistence. In silico analyses identified that saprophytic species possess 40 proteins potentially involved in c-di-GMP signaling, synthesis and degradation (Vasconcelos et al., 2023). This may help these species to survive the environment by fine-tuning their intracellular c-di-GMP levels, allowing them to adapt and respond faster to environment-induced stresses. In addition, some unpublished data from our team also suggest that pathogenic leptospires possess numerous enzymes to regulate their c-di-GMP metabolism. This observation supports our hypothesis that c-di-GMP, by controlling the switch from motile to sessile lifestyle in Leptospira species, is instrumental in the complex lifecycle of pathogenic Leptospira as summarized in Figure 3.
2.3. Impact of floods and climate on leptospirosis epidemiology
Outbreaks of leptospirosis have frequently been associated with floods (Trevejo et al., 1998; Mwachui et al., 2015; Hacker et al., 2020; Phosri, 2022), with evidence that human exposure occurs during or very shortly after flooding events (Togami et al., 2018; Wichapeng et al., 2021). This is in line with the mathematical models developed by Chadsuthi et al. (2021), which predicted that flooding would be a major contributor to disease transmission. This current knowledge suggests that climate change is likely to have a major impact on leptospirosis burden (Lau C. et al., 2010), albeit regional differences (Douchet et al., 2022). In Europe for example, leptospirosis has been identified as the only bacterial zoonosis likely to increase in response to climate change (Dufour et al., 2008). Human leptospirosis was suspected to have increased in the recent years in Europe, e.g., in the Netherlands (Pijnacker et al., 2016), although long-term trends still remain unclear (ECDC, 2022). This flood-association feature drives the epidemiology of massive outbreaks that frequently follow major typhoons or cyclones (Amilasan et al., 2012) or the seasonal monsoon (Schonning et al., 2019; Bierque et al., 2020b). The impact of heavy rainfall on human exposure to environmental contamination with leptospirosis is such that rainfall has been used to decide the implementation of mass chemoprophylaxis (Dechet et al., 2012; Schneider et al., 2017), which however remains a controversial public health strategy. Similarly, climate data have proved relevant to modeling leptospirosis incidence in various settings across different continents (Weinberger et al., 2014; Cucchi et al., 2019; Cunha et al., 2022; Douchet et al., 2022).
2.4. Hypotheses
In a recently published systematic review from our group, we hypothesized that leptospires are washed away from surface soils during heavy rainfall (Bierque et al., 2020b). In this hypothetical scenario supported by the literature review, pathogenic leptospires would mostly survive in soil and be remobilized from surface soils into water bodies by runoff during heavy rain events (Bierque et al., 2020b). Although direct evidence is still lacking, this mechanism would explain the strong link that is widely observed between rainfall and leptospirosis outbreak risk globally. Here again, we hypothesize that leptospires could manage this rapid transition by modulating their c-di-GMP intracellular concentration (Figure 3). If Leptospira species use a biofilm lifestyle in soils, the runoff and induced turbulence may disperse leptospires in a planktonic stage again, possibly prompting a decrease in c-di-GMP intracellular concentration. Of note, this decreased c-di-GMP concentration would not only promote motility (useful for leptospires to find a new habitat), but also virulence that is required to infect new mammal hosts. While saprophytic leptospires are expected to be cleared by the host immune system, pathogenic species may persist in their animal reservoirs for years.
3. Leptospira in the mammalian host
3.1. Infection
3.1.1. Overview
More than 300 serovars of Leptospira have been described to date and can infect virtually any mammal. However, the risk of spreading an environmental pathogen such as Leptospira depends on many factors, ranging from the bacterial load and bacteria's virulence state in the environment to the degree of exposure of different hosts, encompassing the mode and dose of contamination as well as the immune response of the host once infected (Figure 4). Contamination can happen both directly through contact with the urine or kidney tissues of infected reservoir mammals or indirectly through a contaminated water or soil environment (Haake and Levett, 2015; Bierque et al., 2020b; Samrot et al., 2021). It is difficult to estimate the contamination likelihood or the infectious dose because data on Leptospira survival, proliferation, and virulence state in soils are currently unclear. It was estimated that rat colonies, a major reservoir for human contamination, could release over 10 billion leptospires per day (Costa et al., 2015b). A recent meta-analysis also pointed out that the amount of urine produced and the number of individuals in the reservoir populations should also be considered in these estimates (Barragan et al., 2017). Thus, livestock may be more important than rats in environmental human infections in low-income rural Ecuadorian communities. Further, the current hypothesis is that, considering the low densities of leptospires detected in soils (Casanovas-Massana et al., 2018a; Schneider et al., 2018), low environmental infective doses of leptospires might be sufficient to infect a human.
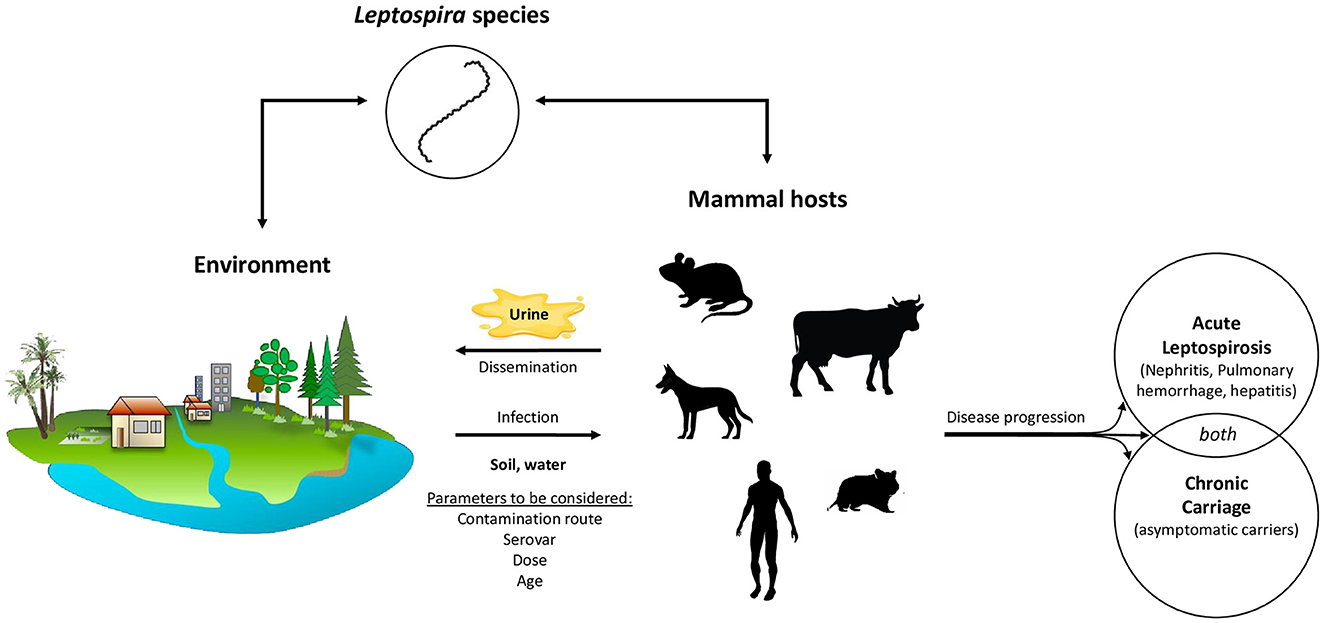
Figure 4. The zoonotic epidemiology of pathogenic Leptospira species is a complex and multifaceted phenomenon. Pathogenic leptospires exhibit a broad host range as they are capable of infecting virtually any mammalian species. The clinical presentation of the resulting infection varies considerably, influenced by a multitude of factors such as the host's immune response, the infectious dose, and the specific Leptospira serovar involved. In some hosts, the infection manifests as an acute syndrome, with symptoms ranging from mild to severe, depending on the ability of the host's immune system to fight the infection and the virulence of the particular Leptospira serovar. Symptoms in severe cases include, kidney damage, liver failure, pulmonary hemorrhage potentially leading to death. However, some hosts remain asymptomatic, showing no signs of illness despite harboring the bacteria. It is important to note that both symptomatic and asymptomatic hosts can become chronic renal carriers of the pathogen. This chronic carriage, wherein the bacteria take up residence in the host's kidneys, can persist throughout the host's entire lifetime which has significant implications for the bacteria environmental excretion. Chronic renal carriers regularly excrete leptospires in their urine, thereby continuously introducing the bacteria back into the environment. This mechanism makes the environment a major reservoir of leptospires, perpetuating the cycle of infection and reinfection, and maintaining the bacteria's presence in the ecosystem.
Among humans, leptospirosis has long been considered an occupational disease affecting sewage workers, farmers, and miners, among others. However, humans can also be infected through water-related recreational activities or sports (swimming, kayaking, camping, …), which are increasingly practiced by people not otherwise at risk for leptospirosis (Monahan A. M. et al., 2009). This risk for leptospirosis can be assessed by quantitative PCR followed by sequencing that allows the detection of pathogenic leptospires in water and soil. Although chemoprophylaxis has been considered to protect humans, data on the effectiveness of such treatment is limited (Guzmán Pérez et al., 2021).
3.1.2. Infection routes
Leptospires are stealth bacteria that actively enter the body through the skin, especially if abraded or cut, and mucous membranes such as conjunctival tissues of the eye (Haake and Levett, 2015). The effect of damaged skin on the probability of infection with leptospires, modeled in hamsters, clearly shows that skin integrity is a crucial immune barrier in protection against leptospires (Gostic et al., 2019). Indeed, abraded skin is almost as efficient as intraperitoneal injection in leading to infection with only hundreds of leptospires. Further, contamination can also happen through the mucosa (notably ocular) pathway. It has also been suggested that the skin and mucosa microbiota associated with cuts, wounds and skin/mucosa lesions may also play a role in leptospires entry into the organism (Vinod Kumar et al., 2019). Normal skin microbiota might also interact with leptospires although such an interaction has not been investigated so far. Regardless of the colonization route, leptospires reach the bloodstream where they have a privileged access to different organs such as the lungs, kidneys, and liver (Goarant et al., 2022), in which they may multiply and cause organ damages. Infected mammals can develop an acute form of leptospirosis, that can be severe, and/or a chronic form of the disease and become carriers of the bacteria. There is increasing evidence that these different forms of the disease are at least partly dependent on the type of immune response mounted by the host (Chin et al., 2018; Vernel-Pauillac and Werts, 2018; Limothai et al., 2021; Petakh et al., 2022). They are also likely to result from the co-evolution of certain hosts and Leptospira serovars, although some reservoir animal species show high diversity of infecting leptospires.
3.1.3. Animal models
Most representations of acute Leptospira infection rely on animal models, particularly hamsters, to mimic infection as occuring in humans or mice for both acute and chronic leptospirosis (Haake, 2006). The infection route often used is the intraperitoneal injection of high doses of bacteria, which is quite different from what is considered the main mode of human infection, i.e., exposure of the skin and mucous membranes to low concentrations of leptospires in the environment. In recent years, subcutaneous infection of hamsters with pathogenic Leptospira has been developed (Villanueva et al., 2014). This model is considered to be more representative of natural animal infection, demonstrating successful infection rates in animals and in some case leading to lethal outcomes (Villanueva et al., 2014).
Other routes of infection have attracted renewed interest (Gomes-Solecki et al., 2017). In mice, it has been shown that the route of inoculation can influence the timing of blood and subsequent organ colonization by pathogenic leptospires. Although infection by swallowing contaminated water has long been the preferred hypothesis in humans, several animal models have emphasized the defensive role played by physical (mucosa) and chemical (saliva and gastric acid) barriers against oral infection by leptospires (Asoh et al., 2014). Moreover, unlike intraperitoneal or transdermal infection, inoculation via the oral mucosa does not result in renal colonization (Nair et al., 2020). Similarly in rats, renal colonization is less frequently observed in the case of inoculation via the skin or mucous membranes (Zilber et al., 2016). In hamsters, the organ damage observed at the time of death was similar when animals were infected with different concentrations of Leptospira. However, at the same dose, infection via the nasal mucosa progressed more slowly and resulted in a lower fatality rate than intraperitoneal infection (Wang et al., 2022). A delay in disease progression was also observed after inoculation via the eye conjunctiva (Wunder et al., 2016). These studies suggest that different routes of infection result in changes in incubation and dissemination kinetics. The maximum Leptospira load in tissue appears independent of the infection route and dose, although the time to reach maximum tissue load depends on both parameters. The duration of the incubation period is directly correlated with the infecting dose in hamsters (Silva et al., 2008) and is thus considered a proxy for the latter (Phraisuwan et al., 2002), as reviewed in Ko et al. (2009). Developing animal models that better reflect the natural infection of humans by leptospires will allow a better understanding of disease progression, as well as a more relevant testing of potential therapeutics and vaccines (Zhang et al., 2012; Zilber et al., 2016; Gostic et al., 2019).
3.2. Immunopathology
Once they have entered the host, leptospires invade the bloodstream and rapidly reach organs, especially lungs, kidneys, and liver. Interestingly, and by contrast with what was initially assumed, both pathogenic and saprophytic leptospires can disseminate in organs in a short-term murine model of infection (Surdel et al., 2022). Humans, as well as other susceptible hosts, first develop a non-specific febrile syndrome, whose clinical presentation is indistinguishable from that of other tropical fevers, notably dengue fever. In roughly 10% of cases, damages of varying degrees of severity to organs particularly targeted by leptospires can occur. Severe leptospirosis is usually characterized by kidney and liver damage and hemorrhages, and patients can die of septic shock with multi-organ failure and/or pulmonary hemorrhages. These data strongly suggest that leptospires, although recognized by the immune system and eliminated from the blood during the acute phase of the infection, can still escape and colonize tissues. This was confirmed by live imaging of a bioluminescent strain in a mouse model of infection (Ratet et al., 2014).
Beyond the physical, chemical, and microbiological barriers of the skin, mucous membranes and secretions, the innate immune system is among the first lines of defense of the organism against potential threats, including pathogenic microorganisms. Not only can antibacterial compounds in the blood, including complement molecules, destroy pathogens but they can also assist in their recognition by scavenger cells. These cells, including but not limited to phagocytes like macrophages (MΦ) and dendritic cells, express pattern recognition receptors (PRRs), which recognize conserved microbial-associated molecular patterns (MAMPs) shared by many bacteria, fungi, protozoa, and viruses (Takeuchi and Akira, 2010). This PRRs/MAMPS recognition triggers the activation of transcription factors such as NF-κB and IRF3, which in turn upregulate genes involved in inflammatory responses, including cytokines, such as interleukin 1β (IL-1β), tumor necrosis factor α (TNF-α) or type I interferons (IFN). Dendritic cells are responsible for the presentation of bacterial antigens to CD4+ T cells, which in turn initiate the adaptive immune response. The quality of PRRs/MAMPs recognition therefore conditions the entire host immune response to the infection.
As currently available data on the innate immune system's uptake of leptospires is limited and often come from in vitro experiments, they may not fully reflect what happens in vivo during infection. However, it has long been known that pathogenic leptospires, unlike saprophytic ones, are resistant to the complement system (Banfi et al., 1982). Moreover, pathogenic leptospires (as other Spirochetes) would be professionals in limiting inflammatory processes, and thus their elimination, notably by escaping recognition by PRRs expressed on the surface of phagocytes (Santecchia et al., 2020). It has also recently been shown that leptospires are even able to enter and exit MΦ and block their cell death (Santecchia et al., 2022; Bonhomme et al., 2023). This recognition also varies significantly depending on the host species infected by Leptospira, which could explain the different levels of susceptibility to infection observed (Bonhomme and Werts, 2022). However, further studies are needed to better characterize the host-specific immune response.
3.3. Hypothesis on the immune response to c-di-GMP
As mentioned before, the virulence status of leptospires, depending on whether they are organized in a biofilm or are planktonic cells, lacks investigation. Microbial nucleic acids, such as single- and double-stranded RNAs or DNAs or cyclic dinucleotides, can all be sensed as MAMPs and elicit an immune reaction that will contribute to successful pathogen elimination (Motwani et al., 2019). However, levels of c-di-GMP in leptospires in vivo in the course of an infection, as well as the detection of c-di-GMP by the host immune system have not yet been studied. We hypothesize that during dissemination in the blood and invasion of the infected host's organs, leptospires are in a highly motile and more virulent planktonic form. Of note, non-motile leptospires have been shown to be non-virulent (Lambert et al., 2012), also supporting a role for c-di-GMP regulation of motility, among other possible regulatory mechanisms, during the infectious process. Accordingly, it is interesting to note that c-di-GMP levels in Leptospira interrogans decrease significantly when shifted from their optimal growth temperature (28–30°C) to a temperature found in the host during infection (37°C) (Xiao et al., 2018). Similarly, these levels decrease when mouse cells are infected in vitro. The hypothetical c-di-GMP regulation during infection is proposed in Figure 3.
Microbial nucleic acids, such as single- and double-stranded RNAs or DNAs or cyclic dinucleotides, can all be sensed as MAMPs and thrive an immune reaction (Motwani et al., 2019). PRRs that recognize cytosolic DNA are Toll-like receptor 9 (TLR9), absent in melanoma 2 (AIM2) and cyclic-GMP–AMP synthase (cGAS). The latter represents the major mode of cytosolic DNA recognition, resulting in the activation of the expression of genes encoding type I interferons (IFN) and in the activation of NF-κB, that are essential for successful pathogen elimination. Briefly, the cGAS/cytosolic DNA binding generates the second messenger cyclic GMP–AMP (cGAMP), which binds to the endoplasmic reticulum (ER)-localized protein STING (stimulator of interferon genes), an essential adaptor in this IFN-dependent response to cytosolic DNA. Interestingly, STING is also able to directly sense cyclic dinucleotides, including c-di-GMP, thereby inducing the IFN response (Burdette et al., 2011). This sensing system probably originated in bacteria, with a defense role against bacteriophages (Morehouse B. R. et al., 2020). Other proteins can recognize c-di-GMP, including the oxidoreductase RECON, leading to the activation of NF-κB and the bacteria removal (McFarland et al., 2017). The topic is still largely unexplored but, considering the capabilities of leptospires to evade PRRs, one could wonder if low levels of c-di-GMP during an acute infection might contribute to Leptospira immune evasion.
4. Chronic carriage in animals and environmental dissemination of Leptospira
4.1. Chronic carriers
Acute leptospirosis is commonly described as a biphasic disease (Haake and Levett, 2015; Gomes-Solecki et al., 2017). In the early stages of the infection, bacteremia occurs within the first days after the incubation period, when leptospires extensively disseminate to all tissues and cause a wide range of clinical symptoms (Athanazio et al., 2008). Immune host response facilitates clearance from almost all host tissues except the brain, eyes, and kidneys where leptospires can survive up to several months (Monahan A. et al., 2009). The infection of a carrier animal is similar in all respects to that of a susceptible host, except that leptospires manage to successfully persist in the renal tubules and animals only show few signs of abnormal histopathology or weight loss (Athanazio et al., 2008; Monahan A. et al., 2009). True reservoirs can thus be defined as asymptomatic infected animals maintaining and actively shedding bacteria, which is essential for persistent disease transmission in the environment (Loureiro and Lilenbaum, 2020). Especially, rats are commonly thought to be the most important sources of Leptospira infection and were identified as asymptomatic carriers of the disease as early as 1917. Intraperitoneal injection of blood, urine or kidney emulsion from asymptomatically infected rats caused death in guinea pigs (Ido et al., 1917), evidencing the virulence and viability of the leptospires carried in their reservoir hosts.
Theoretically, all mammalian species can be chronic carriers and reservoirs, provided they encounter the co-adapted Leptospira strain (Figure 5). For instance, while L. interrogans serovar Lai is highly virulent in humans, it is frequently asymptomatic in mice (Li et al., 2010). Mus musculus mice chronically carry L. borgpetersenii serovar Ballum in their kidneys up to 117 days post infection, without showing any signs of infection (Soupé-Gilbert et al., 2017). Similar observations have been reported for L. interrogans serovar Canicola in dogs, L. interrogans serovar Pomona in pigs, L. borgpetersenii serovar Hardjo and L. interrogans serovar Hardjo in cattle (Monahan A. et al., 2009). The host's immune system is also a factor in determining the host's status as a susceptible or reservoir animal. For instance, there is growing evidence that leptospires are better recognized by innate immune cells in chronic carriers, which might partly explain their rapid clearance from the blood and organs, except within kidney tubules (Vernel-Pauillac and Werts, 2018; Santecchia et al., 2020). Further, several studies showed that newborn mice infected with Leptospira serogroup Icterohaemorrhagiae died while older mice became carriers (Faine, 1962). Similarly, severe disease signs such as jaundice, weight loss and mortality were observed in suckling rats under 2 weeks of age (Muslich et al., 2015). These clinical symptoms mostly disappeared after weaning at 23 days of age, which implies that a mature immune system shall be necessary to become a carrier. Finally, chronic carriage is established during the tubular phase, when bacteria cross kidney tubules towards the lumen, where they are partially protected. The presence of leptospires in host renal tubules was histologically confirmed as early as 1981 (Sterling and Thiermann, 1981). No obvious tissue lesions are detected in the kidney after initial infection, although interstitial nephritis can happen as the infection persists in time (Nally et al., 2005; Athanazio et al., 2008; Monahan et al., 2008; Monahan A. et al., 2009). Antibodies waning over time and serologically negative reservoirs also contributed to postulate that kidney tubules must be immune-privileged to facilitate persistent colonization (Faine et al., 1999; Athanazio et al., 2008; Libonati et al., 2017). However, recent experimental data have shown a sustained IgM production in mice infected with pathogenic leptospires (Vernel-Pauillac et al., 2021). Furthermore, as discussed in Nally et al. (2018), reservoir animals are probably exposed to leptospires very often in the wild, which may maintain their humoral response by contrast to experimentally infected animals. Another possibility is that leptospires are organized in such a way as to remain undetectable by immune cells, or by antibodies directed against them, which are nevertheless present.
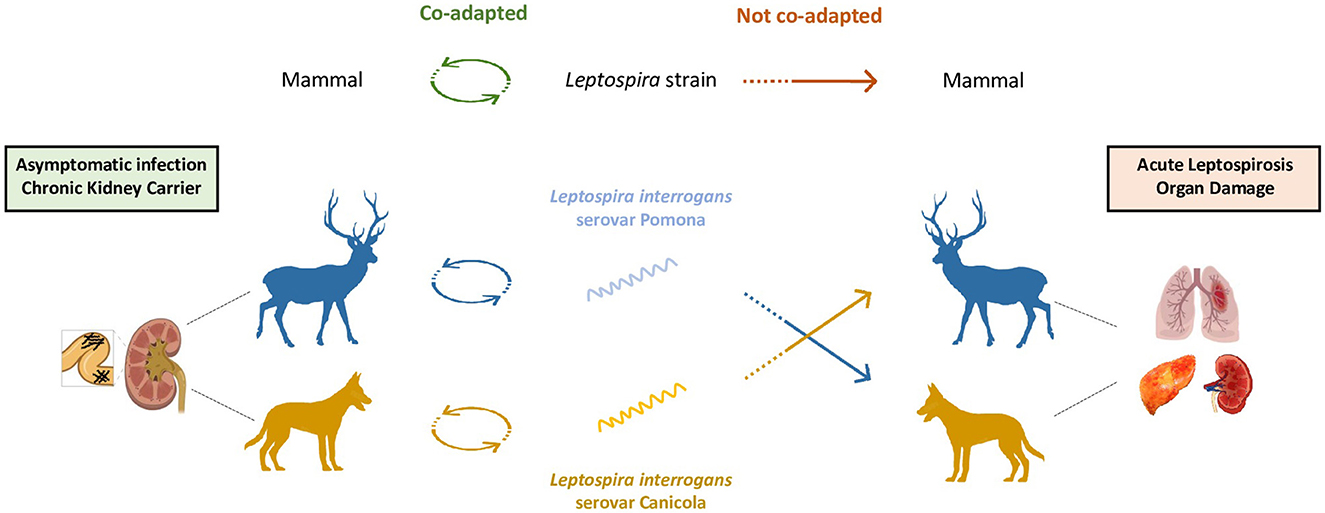
Figure 5. Leptospirosis susceptibility in incidental hosts. Understanding the susceptibility of mammalian hosts to leptospirosis requires a detailed investigation into the relationship between the host and the infecting Leptospira strain. This interaction plays a crucial role in determining the course of the infection and its potential outcomes. In cases in which a mammalian host is co-adapted with its specific infecting strain, the infection tends to follow a benign course (left). The host having evolved alongside the strain is likely to carry the infection asymptomatically. This co-adaptation results in a chronic carriage of the bacteria without manifesting overt disease symptoms, showcasing the intricate balance between the host's immune system and the bacterial strain's virulence. However, when there is no particular co-adaptation between the host and the infecting strain, the infection is more likely to develop into a more severe course (right). In such cases, the host's immune system is ill-equipped to recognize and effectively fight the foreign bacterial strain, resulting in an aggressive infection and potentially severe pathology. The co-adaptation is thought to be influenced by several factors including immune host response's efficiency to recognize the infecting strain, infecting serovar, age of the incidental host and infection route.
4.2. Blood dissemination and renal colonization
Leptospira hematogenous dissemination to the kidneys is an early event that occurs in the very first days upon infection through the glomerulus or peritubular capillaries (Haake and Levett, 2015). The number and motility of Leptospira sp. shall be high enough to overwhelm the natural immune response and blood defenses, allowing few bacteria to reach and colonize the brush border of the proximal renal tubular epithelium (Haake and Levett, 2015; Gomes-Solecki et al., 2017). Although leptospires multiply there during up to 3 weeks post-infection until they fill their niche, they do not colonize new tubules after initial entry (Ratet et al., 2014). Nonetheless, it was hypothesized that the constant level of colonization could be the result of a compensation of the shedding by the multiplication rate in the infected tubules (Ratet et al., 2014).
Hypotheses have been raised as to why leptospires establish in the proximal tubule niche. In addition to being a potentially immune-privileged area for these bacteria, it is also the absorption site of glucose (Mather and Pollock, 2011) and fatty acids (Bobulescu, 2010). Although leptospires do not have an efficient machinery for glucose transportation and metabolism (Zhang et al., 2011), they use fatty acids as their main energy and carbon source for growth (Stalheim, 1966). Leptospires could therefore be provided with enough nutrients to persist and multiply. Importantly, although protected areas, the renal tubules can be a difficult environment for bacteria to live in. Located in the kidneys, the tubular system conducts urine from the glomerulus to the collecting tube and allows, among other things, the reabsorption of a large part of the filtered water as well as the secretion and reabsorption of certain molecules. Thus, a permanent flow passes through this structure and might induce shear forces, which probably constitute a stress for the bacteria that have had to develop strategies to survive and persist in the proximal tubules.
4.3. Biofilm formation and c-di-GMP
Biofilm formation relies on the production of a matrix, the composition of which remains poorly described in Leptospira. To date, we have shown that a significant proportion of the matrix may consist of extracellular DNA (Thibeaux et al., 2020). This component has also been found in the biofilm matrix produced by Leptospira strains isolated from the environment (dos Santos Ribeiro et al., 2023). Its use as a biomarker to confirm the presence of biofilm in vivo in the kidneys of chronically infected animals remains to be investigated and could reinforce current evidence. In 1917, aggregation in renal tubules was observed for the first time, but it was not until 2021 that scanning electron microscopy images and immunohistochemical staining supported the hypothesis of biofilm formation in the renal tubules of Rattus norvegicus (Santos et al., 2021). However, the presence of such structures in vivo remains poorly described. While Brihuega et al. (2012) observed bacterial aggregation in pregnant guinea pigs, Yamaguchi et al. (2018) hypothesized that aggregated leptospires formed a biofilm-like structure during tubular colonization of mice to maintain a protective and replicative niche. Ackermann et al. (2021) recently observed Leptospira biofilm in the vitreous humor of horses with uveitis. It has also been shown that antibiotic administration, specifically doxycycline and enrofloxacin, in chronically infected dogs did not prevent leptospiruria (Mauro and Harkin, 2019). Further treatment with clarithromycin was required to eradicate pathogenic Leptospira from dogs' kidneys. These observations highlight the ability of bacteria in carriers to exhibit resistance to doxycycline and enrofloxacin, which are commonly used as recommended treatment protocols. Another study also demonstrated that an antibiotic protocol commonly employed to treat leptospirosis in rehabilitating California sea lions did not effectively eliminate leptospiruria (Prager et al., 2015). In experimentally infected mice, treatments with penicillin G, ciprofloxacin and azithromycin administered during the chronic phase of leptospirosis did not eradicate leptospires from the kidneys (Ratet et al., 2014). Beyond environmental survival, biofilm formation might therefore also be the strategy adopted by leptospires to resist chemical and mechanical stresses in kidney tubules.
So far, little to nothing is known about c-di-GMP levels by the time of infection or during the establishment of chronic carriage. As mentioned earlier, leptospires need to escape the immune system to colonize the renal tubules, probably by being very motile, and thus having a very low c-di-GMP intracellular concentration. However, once bacteria colonize the renal tubules, they might not need their full motile capacities, and may focus on surviving in the host's tubules and establishing a long-lasting colonization. In this condition, intracellular c-di-GMP levels could rise to signal biofilm formation, a lifestyle best suited for persistence over time. To achieve this goal, leptospires require functional cellular machinery, including a rapid transcriptional capacity to synthesize or degrade c-di-GMP. As mentioned earlier, both pathogenic and saprophytic strains can perform that through regulation of their diguanylate cyclases, phosphodiesterases and hybrid enzymes.
4.4. Excretion of leptospires in the environment
Kidney colonization and maintenance, thought to be promoted by biofilm formation, are key steps to fulfill the bacterial life cycle and its persistence as a zoonotic threat. Once leptospires establish and aggregate in the kidneys, they are excreted by chronic carriers during each micturition event. Notably, infected rats were reported to actively shed more than 106 leptospires/ml via the urine over an extended period of up to 220 days (Costa et al., 2015b). While the bacterial concentration during micturition has often been studied, the physiological state of the leptospires at the time of excretion has received little attention. Urine chemistry, especially pH, which varies depending on the animal species and living conditions, seems to influence Leptospira physiology upon excretion (Nau et al., 2020). In addition, the way in which leptospires enter the environment may be crucial to their survival. Are the bacteria excreted in biofilm form, planktonic form, or aggregates?
When a primary urine stream passes through the kidneys of carrier animals, it is possible that small aggregates of leptospires are detached and temporarily stored in the bladder before being excreted with a much larger stream into the environment. However, to date very little information is available on the virulence, motility, or biofilm-forming capacity of these leptospires. A proteomic study conducted in 2008 on R. norvegicus urine showed that leptospires excreted from renal tubules regulate their protein and antigen expression, which reduces opsonization by the host's antibodies (Monahan et al., 2008). During infection with leptospires and in response to a mammalian host immunity, numerous post-translational modifications are initiated such as modifications in lysine residues from LipL32 (Witchell et al., 2014) or changes in lipopolysaccharide O antigen (Nally et al., 2005). These modifications were postulated to have a role in the interaction with the host, in the virulence and in the renal colonization (Nally et al., 2017), but their maintenance and usefulness during bacterial release is not yet known. A comparative study with the proteome of a biofilm culture could highlight the specificities of each lifestyle and point out the proteins and post-translational modifications required for a transition from the aggregate state in the kidney to the planktonic state in the urine.
Cyclic-di-GMP levels during this transition are not properly described and can only be hypothesized. As mentioned earlier, when bacteria return to a planktonic form, the intracellular c-di-GMP level decreases to allow them to regain their motility and attempt to colonize new environments or hosts. However, when the transition to planktonic form is forced by an external event such as the urine flow, similarly to what happens when leptospires are washed off by heavy rainfall events, the response of this second messenger remains unknown. One important question arising from these considerations is whether c-di-GMP concentration decreases to allow a return to the planktonic state or increases to allow the detached piece of biofilm to keep on expanding. One could hypothesize that both processes may occur depending on where the infected urine is excreted. Further data on this topic would complement our current knowledge on the complex life cycle of leptospires by helping to explain their persistence in the environment.
5. Conclusion: synthesis, research gaps and way forward
In this review, we described the biological life cycle of pathogenic leptospires in real life conditions. We notably focused on the transition phases of this complex life cycle, which may represent major challenges for leptospires in terms of need to rapidly adapt to a new biological or abiotic environment. We proposed some hypotheses on the possible role of transitioning between biofilm or planktonic lifestyle, a mechanism regulated by the second intracellular messenger c-di-GMP as a clue to manage these transitions. We propose to take better account of pathogenic Leptospira lifestyle at each stage of their cycle to better understand the eco-epidemiology of the disease they cause. In this regard, secondary cyclic messengers, notably c-di-GMP, quantification at all stages of this cycle may be a determining approach to better understand Leptospira strategies. Gaining a better understanding of Leptospira biofilm in natura and of the microbiota supporting Leptospira environmental survival might also enlighten our understanding of the disease in a holistic approach. Biofilm has also been evidenced in some immune-privileged sites of chronically infected hosts, opening a new field of research, including topics of host-pathogen interactions and immune evasion strategies. Finally, how Leptospira- c-di-GMP triggers the immune system should also be further considered in future investigations.
Author contributions
Conceptualization and writing-original draft preparation: GD, JC, RT, and CG. Writing-review and editing: GD, JC, FJ, MP, RT, and CG. Supervision: FJ, PG, PG-G, and CG. Project administration and funding acquisition: FJ, PG, PG-G, MP, and CG. All authors have substantially contributed and validated the final manuscript and agreed to the published version of the manuscript.
Funding
This research was funded by The French National Research Agency, grant number SPIraL-19-CE35-0006-01 and The Consortium for the Research Higher Education and Innovation in New Caledonia, CRESICA, Grant, ASSURPLUHYT.
Acknowledgments
Acknowledgments are due to scientific colleagues and partners for contributing to our scientific discussions, especially Monika Lemestre, Chloé Chavoix, Rodrigue Govan, Audrey Leopold through her ValoPro-NC project.
Conflict of interest
The authors declare that the research was conducted in the absence of any commercial or financial relationships that could be construed as a potential conflict of interest.
Publisher's note
All claims expressed in this article are solely those of the authors and do not necessarily represent those of their affiliated organizations, or those of the publisher, the editors and the reviewers. Any product that may be evaluated in this article, or claim that may be made by its manufacturer, is not guaranteed or endorsed by the publisher.
References
Ackermann, K., Kenngott, R., Settles, M., Gerhards, H., Maierl, J., Wollanke, B., et al. (2021). In vivo biofilm formation of pathogenic Leptospira spp. in the vitreous humor of horses with recurrent uveitis. Microorganisms 9, 1915. doi: 10.3390/microorganisms9091915
Amilasan, A. T., Ujiie, M., Suzuki, M., Salva, E., Belo, M. C. P., Koizumi, N., et al. (2012). Outbreak of leptospirosis after flood, the Philippines, 2009. Emerging Infect. Dis. 18, 91–94. doi: 10.3201/eid1801.101892
Asoh, T., Saito, M., Villanueva, S. Y., Kanemaru, T., Gloriani, N., Yoshida, S., et al. (2014). Natural defense by saliva and mucosa against oral infection by Leptospira. Can. J. Microbiol. 60, 383–389. doi: 10.1139/cjm-2014-0016
Athanazio, D. A., Silva, E. F., Santos, C. S., Rocha, G. M., Vannier-Santos, M. A., McBride, A. J., et al. (2008). Rattus norvegicus as a model for persistent renal colonization by pathogenic Leptospira interrogans. Acta Trop. 105, 176–180. doi: 10.1016/j.actatropica.2007.10.012
Banfi, E., Cinco, M., Bellini, M., and Soranzo, M. R. (1982). The role of antibodies and serum complement in the interaction between macrophages and leptospires. J. Gen. Microbiol. 128, 813–816. doi: 10.1099/00221287-128-4-813
Barragan, V., Nieto, N., Keim, P., and Pearson, T. (2017). Meta-analysis to estimate the load of Leptospira excreted in urine: beyond rats as important sources of transmission in low-income rural communities. BMC Res. Notes. 10, 71. doi: 10.1186/s13104-017-2384-4
Bierque, E., Soupé-Gilbert, M. E., Thibeaux, R., Girault, D., Guentas, L., Goarant, C., et al. (2020a). Leptospira interrogans retains direct virulence after long starvation in water. Curr. Microbiol. 77, 3035–3043. doi: 10.1007/s00284-020-02128-7
Bierque, E., Thibeaux, R., Girault, D., Soupé-Gilbert, M. E., and Goarant, C. A. (2020b). systematic review of Leptospira in water and soil environments. PLoS ONE 15, e0227055. doi: 10.1371/journal.pone.0227055
Bobulescu, I. A. (2010). Renal lipid metabolism and lipotoxicity. Curr. Opin. Nephrol. Hypertens. 19, 393–402. doi: 10.1097/MNH.0b013e32833aa4ac
Bonhomme, D., Hernandez-Trejo, V., Papadopoulos, S., Pigache, R., Fanton d'Andon, M., Outlioua, A., et al. (2023). Leptospira interrogans prevents macrophage cell death and pyroptotic IL-1β release through its atypical lipopolysaccharide. J. Immunol. 210, 459–474. doi: 10.4049/jimmunol.2200584
Bonhomme, D., and Werts, C. (2022). Host and species-specificities of pattern recognition receptors upon infection with Leptospira interrogans. Front. Cell. Infect. Microbiol. 12, 932137. doi: 10.3389/fcimb.2022.932137
Brihuega, B., Samartino, L., Auteri, C., Venzano, A., and Caimi, K. (2012). In vivo cell aggregations of a recent swine biofilm-forming isolate of Leptospira interrogans strain from Argentina. Rev. Argent. Microbiol. 44, 138–143.
Bulach, D. M., Zuerner, R. L., Wilson, P., Seemann, T., McGrath, A., Cullen, P. A., et al. (2006). Genome reduction in Leptospira borgpetersenii reflects limited transmission potential. Proc. Natl. Acad. Sci. USA. 103, 14560–14565. doi: 10.1073/pnas.0603979103
Burdette, D. L., Monroe, K. M., Sotelo-Troha, K., Iwig, J. S., Eckert, B., Hyodo, M., et al. (2011). STING is a direct innate immune sensor of cyclic di-GMP. Nature 478, 515–518. doi: 10.1038/nature10429
Casanovas-Massana, A., Costa, F., Riediger, I. N., Cunha, M., de Oliveira, D., Mota, D. C., et al. (2018a). Spatial and temporal dynamics of pathogenic Leptospira in surface waters from the urban slum environment. Water Res. 130, 176–184. doi: 10.1016/j.watres.2017.11.068
Casanovas-Massana, A., Pedra, G. G., Wunder, E. A. Jr., Diggle, P. J., Begon, M., and Ko, A. I. (2018b). Quantification of Leptospira interrogans survival in soil and water microcosms. Appl. Environ. Microbiol. 84, e00507-18. doi: 10.1128/AEM.00507-18
Chadsuthi, S., Chalvet-Monfray, K., Wiratsudakul, A., and Modchang, C. (2021). The effects of flooding and weather conditions on leptospirosis transmission in Thailand. Sci. Rep. 11, 1486. doi: 10.1038/s41598-020-79546-x
Chiani, Y. A.-O., Jacob, P., Mayora, G., Aquino, D. S., Quintana, R. D., Mesa, L., et al. (2023). Presence of Leptospira spp. in a mosaic of wetlands used for livestock raising under differing hydroclimatic conditions. Appl. Environ. Microbiol. 89, e0197122. doi: 10.1128/aem.01971-22
Chin, V. K., Ty, L., Lim, W. F., Wan Shahriman, Y. W. Y., An, S., Zamberi, S., et al. (2018). Leptospirosis in human: biomarkers in host immune responses. Microbiol. Res. 207, 108–115. doi: 10.1016/j.micres.2017.11.015
Costa, F., Hagan, J. E., Calcagno, J., Kane, M., Torgerson, P., Martinez-Silveira, M. S., et al. (2015a). Global morbidity and mortality of leptospirosis: a systematic review. PLoS Negl. Trop. Dis. 9, e0003898. doi: 10.1371/journal.pntd.0003898
Costa, F., Ribeiro, G. S., Felzemburgh, R. D. M., Santos, N., Reis, R. B., Santos, A. C., et al. (2014). Influence of household rat infestation on Leptospira transmission in the urban slum environment. PLoS Negl. Trop. Dis. 8, e3338. doi: 10.1371/journal.pntd.0003338
Costa, F., Wunder, E. A. Jr., De Oliveira, D., Bisht, V., Rodrigues, G., Reis, M. G., et al. (2015b). Patterns in Leptospira shedding in Norway rats (Rattus norvegicus) from Brazilian slum communities at high risk of disease transmission. PLoS Negl. Trop. Dis. 9, e0003819. doi: 10.1371/journal.pntd.0003819
Cucchi, K., Liu, R., Collender, P. A., Cheng, Q., Li, C., Hoover, C. M., et al. (2019). Hydroclimatic drivers of highly seasonal leptospirosis incidence suggest prominent soil reservoir of pathogenic Leptospira spp. in rural western China. PLoS Negl. Trop. Dis. 13, e0007968. doi: 10.1371/journal.pntd.0007968
Cunha, M., Costa, F., Ribeiro, G. S., Carvalho, M. S., Reis, R. B., Nery, N. Jr., et al. (2022). Rainfall and other meteorological factors as drivers of urban transmission of leptospirosis. PLoS Negl. Trop. Dis. 16, e0007507. doi: 10.1371/journal.pntd.0007507
Dechet, A. M., Parsons, M., Rambaran, M., Mohamed-Rambaran, P., Florendo-Cumbermack, A., Persaud, S., et al. (2012). Leptospirosis outbreak following severe flooding: a rapid assessment and mass prophylaxis campaign; Guyana, January-February 2005. PLoS ONE 7, e39672. doi: 10.1371/journal.pone.0039672
dos Santos Ribeiro, P., Carvalho, N. B., Aburjaile, F., Sousa, T., Veríssimo, G., Gomes, T., et al. (2023). Environmental biofilms from an urban community in Salvador, Brazil, shelter previously uncharacterized saprophytic Leptospira. Microb. Ecol. doi: 10.1007/s00248-023-02253-3 [Epub ahead of print].
Douchet, L., Goarant, C., Mangeas, M., Menkes, C., Hinjoy, S., Herbreteau, V., et al. (2022). Unraveling the invisible leptospirosis in mainland Southeast Asia and its fate under climate change. Sci. Total Environ. 832, 15518. doi: 10.1016/j.scitotenv.2022.155018
Dufour, B., Moutou, F., Hattenberger, A. M., and Rodhain, F. (2008). Global change: impact, management, risk approach and health measures–the case of Europe. Rev. Off. Int. Epizoot. 27, 529–550. doi: 10.20506/rst.27.2.1817
ECDC (2022). Leptospirosis - Annual Epidemiological Report for 2018. Stockholm: European Centre for Disease Prevention and Control; 2018 Octobre 2022.
Faine, S. (1962). Factors affecting the development of the carrier state in leptospirosis. J. Hyg. 60, 427–434. doi: 10.1017/S0022172400020556
Faine, S., Adler, B., Bolin, C., and Perolat, P. (1999). Leptospira and Leptospirosis, 2nd ed. (MedSci, ed). Melbourne, VIC: MedSci, 272.
Fernandes, L. G. V., Stone, N. E., Roe, C. C., Goris, M. G. A., van der Linden, H., Sahl, J. W., et al. (2022). Leptospira sanjuanensis sp. nov., a pathogenic species of the genus Leptospira isolated from soil in Puerto Rico. Int. J. Syst. Evol. Microbiol. 72. doi: 10.1099/ijsem.0.005560
Ganoza, C. A., Matthias, M. A., Collins-Richards, D., Brouwer, K. C., Cunningham, C. B., Segura, E. R., et al. (2006). Determining risk for severe leptospirosis by molecular analysis of environmental surface waters for pathogenic Leptospira. PLoS Med. 3, e308. doi: 10.1371/journal.pmed.0030308
Goarant, C., Adler, B., and De la Pena Moctezuma, A. (2022). “Leptospira,” in Pathogenesis of Bacterial Infections in Animals, 5th ed., eds J. F. Prescott, J. Boyce, J. I. MacInnes, A. N. Rycroft, F. V. Immerseel, and J. A. Vázquez-Boland (Hoboken, NJ: Wiley Blackwell), 502–27. doi: 10.1002/9781119754862.ch23
Goarant, C., Picardeau, M., Morand, S., and McIntyre, K. M. (2019). Leptospirosis under the bibliometrics radar: evidence for a vicious circle of neglect. J. Glob. Health. 9, 010302. doi: 10.7189/jogh.09.010302
Gomes-Solecki, M., Santecchia, I., and Werts, C. (2017). Animal models of leptospirosis: of mice and hamsters. Front. Immunol. 8, 58. doi: 10.3389/fimmu.2017.00058
Gostic, K. M., Wunder, E. A. Jr., Bisht, V., Hamond, C., Julian, T. R., Ko, A. I., et al. (2019). Mechanistic dose-response modelling of animal challenge data shows that intact skin is a crucial barrier to leptospiral infection. Philos. Trans. R. Soc. Lond. B. Biol. Sci. 374, 20190367. doi: 10.1098/rstb.2019.0367
Guzmán Pérez, M., Blanch Sancho, J. J., Segura Luque, J. C., Mateos Rodriguez, F., Martínez Alfaro, E., and Solís García Del Pozo, J. (2021). Current evidence on the antimicrobial treatment and chemoprophylaxis of human leptospirosis: a meta-analysis. Pathogens 10, 1125. doi: 10.3390/pathogens10091125
Haake, D. A. (2006). Hamster model of leptospirosis. Curr. Protoc. Microbiol. Chapter 12, Unit 12E 2. doi: 10.1002/9780471729259.mc12e02s02
Haake, D. A., and Levett, P. N. (2015). Leptospirosis in humans. Curr. Top. Microbiol. Immunol. 387, 65–97. doi: 10.1007/978-3-662-45059-8_5
Hacker, K. P., Sacramento, G. A., Cruz, J. S., de Oliveira, D., Nery, N. Jr., Lindow, J. C., et al. (2020). Influence of rainfall on Leptospira infection and disease in a tropical urban setting, Brazil. Emerging Infect. Dis. 26, 311–314. doi: 10.3201/eid2602.190102
Haselkorn, T. S., Jimenez, D., Bashir, U., Sallinger, E., Queller, D. C., Strassmann, J. E., et al. (2021). Novel Chlamydiae and Amoebophilus endosymbionts are prevalent in wild isolates of the model social amoeba Dictyostelium discoideum. Environ. Microbiol. Rep. 13, 708–719. doi: 10.1111/1758-2229.12985
Hellstrom, J. S., and Marshall, R. B. (1978). Survival of Leptospira interrogans serovar pomona in an acidic soil under simulated New Zealand field conditions. Res. Vet. Sci. 25, 29–33. doi: 10.1016/S0034-5288(18)33003-0
Hengge, R. (2009). Principles of c-di-GMP signalling in bacteria. Nat. Rev. Microbiol. 7, 263–273. doi: 10.1038/nrmicro2109
Henry, R. A., and Johnson, R. C. (1978). Distribution of the genus Leptospira in soil and water. Appl. Environ. Microbiol. 35, 492–499. doi: 10.1128/aem.35.3.492-499.1978
Hickman, J. W., Tifrea, D. F., and Harwood, C. S. (2005). A chemosensory system that regulates biofilm formation through modulation of cyclic diguanylate levels. Proc. Natl. Acad. Sci. USA. 102, 14422–14427. doi: 10.1073/pnas.0507170102
Hong, H., Chen, S., Fang, Q., Algeo, T. J., and Zhao, L. (2019). Adsorption of organic matter on clay minerals in the Dajiuhu peat soil chronosequence, South China. Appl. Clay Sci. 178, 105125. doi: 10.1016/j.clay.2019.105125
Hornsby, R. L., Alt, D. P., and Nally, J. E. (2020). Isolation and propagation of leptospires at 37 °C directly from the mammalian host. Sci. Rep. 10, 9620. doi: 10.1038/s41598-020-66526-4
Ido, Y., Hoki, R., Ito, H., and Wani, H. (1917). The rat as a carrier of Spirochaeta Icterohaemorrhaguae, the causative agent of Weil's disease (Spirochaetosis Icterohaemorrhagica). J. Exp. Med. 26, 341–353. doi: 10.1084/jem.26.3.341
Iraola, G., Spangenberg, L., Lopes Bastos, B., Graña, M., Vasconcelos, L., Almeida, Á., et al. (2016). Transcriptome sequencing reveals wide expression reprogramming of basal and unknown genes in leptospira biflexa biofilms. mSphere. 1, e00042–16. doi: 10.1128/mSphere.00042-16
Islam, M. R., Singh, B., and Dijkstra, F. A. (2022). Stabilisation of soil organic matter: interactions between clay and microbes. Biogeochemistry 160, 145–158. doi: 10.1007/s10533-022-00956-2
Jenal, U., Reinders, A., and Lori, C. (2017). Cyclic di-GMP: second messenger extraordinaire. Nat. Rev. 15, 271–284. doi: 10.1038/nrmicro.2016.190
Ko, A. I., Goarant, C., and Picardeau, M. (2009). Leptospira: the dawn of the molecular genetics era for an emerging zoonotic pathogen. Nat. Rev. Microbiol. 7, 736–747. doi: 10.1038/nrmicro2208
Kumar, K. V., Lall, C., Raj, R. V., Vedhagiri, K., and Vijayachari, P. (2015). Coexistence and survival of pathogenic leptospires by formation of biofilm with Azospirillum. FEMS Microbiol. Ecol. 91, fiv051. doi: 10.1093/femsec/fiv051
Kurilung, A., Keeratipusana, C., Horiike, T., Suriyaphol, P., Hampson, D. J., Prapasarakul, N., et al. (2019). Chronology of emergence of the genus Leptospira and over-representation of gene families enriched by vitamin B2, B12 biosynthesis, cell adhesion and external encapsulating structure in L. interrogans isolates from asymptomatic dogs. Infect. Genet. Evol. 73, 1–12. doi: 10.1016/j.meegid.2019.04.005
Lall, C., Vinod Kumar, K., Raj, R. V., Vedhagiri, K., Sunish, I. P., Vijayachari, P., et al. (2018). Correlation between physicochemical properties of soil and presence of Leptospira. Ecohealth 15, 670–675. doi: 10.1007/s10393-018-1346-1
Lambert, A., Picardeau, M., Haake, D. A., Sermswan, R. W., Srikram, A., Adler, B., et al. (2012). FlaA proteins in Leptospira interrogans are essential for motility and virulence, but not required for the formation of flagella sheath. Infect. Immun. 80, 2019–2025. doi: 10.1128/IAI.00131-12
Lau, C., Smythe, L., and Weinstein, P. (2010). Leptospirosis: an emerging disease in travellers. Travel Med. Infect. Dis. 8, 33–39. doi: 10.1016/j.tmaid.2009.12.002
Lau, C. L., Smythe, L. D., Craig, S. B., and Weinstein, P. (2010). Climate change, flooding, urbanisation and leptospirosis: fuelling the fire? Trans. R. Soc. Trop. Med. Hyg. 104, 631–638. doi: 10.1016/j.trstmh.2010.07.002
Li, S., Ojcius, D. M., Liao, S., Li, L., Xue, F., Dong, H., et al. (2010). Replication or death: distinct fates of pathogenic Leptospira strain Lai within macrophages of human or mouse origin. Innate Immun. 16, 80–92. doi: 10.1177/1753425909105580
Libonati, H., Pinto, P. S., and Lilenbaum, W. (2017). Seronegativity of bovines face to their own recovered leptospiral isolates. Microb. Pathog. 108, 101–103. doi: 10.1016/j.micpath.2017.05.001
Limothai, U., Lumlertgul, N., Sirivongrangson, P., Kulvichit, W., Tachaboon, S., Dinhuzen, J., et al. (2021). The role of leptospiremia and specific immune response in severe leptospirosis. Sci. Rep. 11, 14630. doi: 10.1038/s41598-021-94073-z
Loureiro, A. P., and Lilenbaum, W. (2020). Genital bovine leptospirosis: a new look for an old disease. Theriogenology 141, 41–47. doi: 10.1016/j.theriogenology.2019.09.011
Mather, A., and Pollock, C. (2011). Glucose handling by the kidney. Kidney Int. Suppl. 120, S1–6. doi: 10.1038/ki.2010.509
Mauro, T., and Harkin, K. (2019). Persistent leptospiruria in five dogs despite antimicrobial treatment (2000-2017). J. Am. Anim. Hosp. Assoc. 55, 42–47. doi: 10.5326/JAAHA-MS-6882
McFarland, A. P., Luo, S., Ahmed-Qadri, F., Zuck, M., Thayer, E. F., Goo, Y. A., et al. (2017). Sensing of bacterial cyclic dinucleotides by the oxidoreductase RECON promotes NF-κB activation and shapes a proinflammatory antibacterial state. Immunity 46, 433–445. doi: 10.1016/j.immuni.2017.02.014
Meganathan, Y., Vishwakarma, A., and Ramya, M. (2022). Biofilm formation and social interaction of Leptospira in natural and artificial environments. Res. Microbiol. 173, 103981. doi: 10.1016/j.resmic.2022.103981
Miller, E., Barragan, V., Chiriboga, J., Weddell, C., Luna, L., Jiménez, D. J., et al. (2021). Leptospira in river and soil in a highly endemic area of Ecuador. BMC Microbiol. 21, 17. doi: 10.1186/s12866-020-02069-y
Monahan, A., Callanan, J., and Nally, J. (2009). Host-pathogen interactions in the kidney during chronic leptospirosis. Vet. Pathol. 46, 792–799. doi: 10.1354/vp.08-VP-0265-N-REV
Monahan, A. M., Callanan, J. J., and Nally, J. E. (2008). Proteomic analysis of Leptospira interrogans shed in urine of chronically infected hosts. Infect. Immun. 76, 4952–4958. doi: 10.1128/IAI.00511-08
Monahan, A. M., Miller, I. S., and Nally, J. E. (2009). Leptospirosis: risks during recreational activities. J. Appl. Microbiol. 107, 707–713. doi: 10.1111/j.1365-2672.2009.04220.x
Morehouse, B. R., Govande, A. A., Millman, A., Keszei, A. F. A., Lowey, B., Ofir, G., et al. (2020). STING cyclic dinucleotide sensing originated in bacteria. Nature 586, 429–433. doi: 10.1038/s41586-020-2719-5
Motwani, M., Pesiridis, S., and Fitzgerald, K. A. (2019). DNA sensing by the cGAS-STING pathway in health and disease. Nat. Rev. Genet. 20, 657–674. doi: 10.1038/s41576-019-0151-1
Muslich, L. T., Villanueva, S. Y. A. M., Amran, M. Y., Segawa, T., Saito, M., Yoshida, S.-I., et al. (2015). Characterization of Leptospira infection in suckling and weaning rat pups. Comp. Immunol. Microbiol. Infect. Dis. 38, 47–55. doi: 10.1016/j.cimid.2014.11.001
Mwachui, M. A., Crump, L., Hartskeerl, R., Zinsstag, J., and Hattendorf, J. (2015). Environmental and behavioural determinants of leptospirosis transmission: a systematic review. PLoS Negl. Trop. Dis. 9, e0003843. doi: 10.1371/journal.pntd.0003843
Nair, N., Guedes, M. S., Werts, C., and Gomes-Solecki, M. (2020). The route of infection with Leptospira interrogans serovar Copenhageni affects the kinetics of bacterial dissemination and kidney colonization. PLoS Negl. Trop. Dis. 14, e0007950. doi: 10.1371/journal.pntd.0007950
Nally, J. E., Chow, E., Fishbein, M. C., Blanco, D. R., and Lovett, M. A. (2005). Changes in lipopolysaccharide O antigen distinguish acute versus chronic Leptospira interrogans infections. Infect. Immun. 73, 3251–3260. doi: 10.1128/IAI.73.6.3251-3260.2005
Nally, J. E., Grassmann, A. A., Planchon, S., Sergeant, K., Renaut, J., Seshu, J., et al. (2017). Pathogenic leptospires modulate protein expression and post-translational modifications in response to mammalian host signals. Front. Cell. Infect. Microbiol. 7, 362. doi: 10.3389/fcimb.2017.00362
Nally, J. E., Wilson-Welder, J. H., Hornsby, R. L., Palmer, M. V., and Alt, D. P. (2018). Inbred rats as a model to study persistent renal leptospirosis and associated cellular immune responsiveness. Front. Cell. Infect. Microbiol. 8, 66. doi: 10.3389/fcimb.2018.00066
Nau, L. H., Obiegala, A., Król, N., Mayer-Scholl, A., and Pfeffer, M. (2020). Survival time of Leptospira kirschneri serovar Grippotyphosa under different environmental conditions. PLoS ONE. 15, e0236007. doi: 10.1371/journal.pone.0236007
Okazaki, W., and Ringen, L. M. (1957). Some effects of various environmental conditions on the survival of Leptospira pomona. Am. J. Vet. Res. 18, 219–223.
Parker, J., and Walker, M. (2011). Survival of a pathogenic Leptospira serovar in response to combined in vitro pH and temperature stresses. Vet. Microbiol. 152, 146–150. doi: 10.1016/j.vetmic.2011.04.028
Petakh, P. A.-O., Isevych, V., Kamyshnyi, A. A.-O., and Oksenych, V. A.-O. (2022). Weil's disease-immunopathogenesis, multiple organ failure, and potential role of gut microbiota. Biomolecules 12, 1830. doi: 10.3390/biom12121830
Phosri, A. (2022). Effects of rainfall on human leptospirosis in Thailand: evidence of multi-province study using distributed lag non-linear model. Stoch. Environ. Res. Risk Assess. 36, 4119–4132. doi: 10.1007/s00477-022-02250-x
Phraisuwan, P., Whitney, E. A., Tharmaphornpilas, P., Guharat, S., Thongkamsamut, S., Aresagig, S., et al. (2002). Leptospirosis: skin wounds and control strategies, Thailand, 1999. Emerging Infect. Dis. 8, 1455–1459. doi: 10.3201/eid0812.020180
Pijnacker, R., Goris, M. G., Te Wierik, M. J., Broens, E. M., van der Giessen, J. W., de Rosa, M., et al. (2016). Marked increase in leptospirosis infections in humans and dogs in the Netherlands, 2014. Euro. Surveill. 21. doi: 10.2807/1560-7917.ES.2016.21.17.30211
Prager, K. C., Alt, D. P., Buhnerkempe, M. G., Greig, D. J., Galloway, R. L., Wu, Q., et al. (2015). Antibiotic efficacy in eliminating leptospiruria in california sea lions (zalophus californianus) stranding with leptospirosis. Aquat Mamm. 41, 203–212. doi: 10.1578/AM.41.2.2015.203
Ratet, G., Veyrier, F. J., Fanton d'Andon, M., Kammerscheit, X., Nicola, M.-A., Picardeau, M., et al. (2014). Live imaging of bioluminescent Leptospira interrogans in mice reveals renal colonization as a stealth escape from the blood defenses and antibiotics. PLoS Negl. Trop. Dis. 8, e3359. doi: 10.1371/journal.pntd.0003359
Ristow, P., Bourhy, P., Kerneis, S., Schmitt, C., Prevost, M. C., Lilenbaum, W., et al. (2008). Biofilm formation by saprophytic and pathogenic leptospires. Microbiology 154(Pt 5), 1309–1317. doi: 10.1099/mic.0.2007/014746-0
Ryjenkov, D. A., Tarutina, M., Moskvin, O. V., and Gomelsky, M. (2005). Cyclic diguanylate is a ubiquitous signaling molecule in bacteria: insights into biochemistry of the GGDEF protein domain. J. Bacteriol. 187, 1792–1798. doi: 10.1128/JB.187.5.1792-1798.2005
Saito, M., Villanueva, S. Y., Chakraborty, A., Miyahara, S., Segawa, T., Asoh, T., et al. (2013). Comparative analysis of Leptospira strains isolated from environmental soil and water in the Philippines and Japan. Appl. Environ. Microbiol. 79, 601–609. doi: 10.1128/AEM.02728-12
Samrot, A. A.-O., Sean, T. A.-O., Bhavya, K. S., Sahithya, C. S., Chan-Drasekaran, S., Palanisamy, R., et al. (2021). Leptospiral infection, pathogenesis and its diagnosis-a review. Pathogens 10, 145. doi: 10.3390/pathogens10020145
Santecchia, I., Bonhomme, D., Papadopoulos, S., Escoll, P., Giraud-Gatineau, A., Moya-Nilges, M., et al. (2022). Alive pathogenic and saprophytic leptospires enter and exit human and mouse macrophages with no intracellular replication. Front. Cell. Infect. Microbiol. 12, 936931. doi: 10.3389/fcimb.2022.936931
Santecchia, I., Ferrer, M. F., Vieira, M. L., Gómez, R. M., and Werts, C. (2020). Phagocyte escape of Leptospira: the role of TLRs and NLRs. Front. Immunol. 11, 571816. doi: 10.3389/fimmu.2020.571816
Santos, A. A. N., Ribeiro, P. D. S., da França, G. V., Souza, F. N., Ramos, E. A. G., Figueira, C. P., et al. (2021). Leptospira interrogans biofilm formation in Rattus norvegicus (Norway rats) natural reservoirs. PLoS Negl. Trop. Dis. 15, e0009736. doi: 10.1371/journal.pntd.0009736
Sato, Y., Mizuyama, M., Sato, M., Minamoto, T., Kimura, R., Toma, C., et al. (2019). Environmental DNA metabarcoding to detect pathogenic Leptospira and associated organisms in leptospirosis-endemic areas of Japan. Sci. Rep. 9, 6575. doi: 10.1038/s41598-019-42978-1
Schmidt, A. J., Ryjenkov, D. A., and Gomelsky, M. (2005). The ubiquitous protein domain EAL is a cyclic diguanylate-specific phosphodiesterase: enzymatically active and inactive EAL domains. J. Bacteriol. 187, 4774–4781. doi: 10.1128/JB.187.14.4774-4781.2005
Schmidt, M. P., and Martínez, C. E. (2016). Kinetic and conformational insights of protein adsorption onto montmorillonite revealed using in situ ATR-FTIR/2D-COS. Langmuir 32, 7719–7729. doi: 10.1021/acs.langmuir.6b00786
Schneider, A. G., Casanovas-Massana, A., Hacker, K. P., Wunder, E. A. Jr., Begon, M., Reis, M. G., et al. (2018). Quantification of pathogenic Leptospira in the soils of a Brazilian urban slum. PLoS Negl. Trop. Dis. 12, e0006415. doi: 10.1371/journal.pntd.0006415
Schneider, M. C., Velasco-Hernandez, J., Min, K. D., Leonel, D. G., Baca-Carrasco, D., Gompper, M. E., et al. (2017). The use of chemoprophylaxis after floods to reduce the occurrence and impact of leptospirosis outbreaks. Int. J. Environ. Res. Public Health 14, 594. doi: 10.3390/ijerph14060594
Schonning, M. H., Phelps, M. D., Warnasekara, J., Agampodi, S. B., and Furu, P. A. (2019). Case-control study of environmental and occupational risks of leptospirosis in Sri Lanka. Ecohealth 16, 534–543. doi: 10.1007/s10393-019-01448-w
Scialfa, E., Grune, S., Brihuega, B., Aguirre, P., and Rivero, M. (2018). Isolation of saprophytic Leptospira spp. from a selected environmental water source of Argentina. Rev. Argent. Microbiol. 50, 323–326. doi: 10.1016/j.ram.2017.08.003
Silva, E. F., Santos, C. S., Athanazio, D. A., Seyffert, N., Seixas, F. K., Cerqueira, G. M., et al. (2008). Characterization of virulence of Leptospira isolates in a hamster model. Vaccine 26, 3892–3896. doi: 10.1016/j.vaccine.2008.04.085
Smith, C. E., Turner, L. H., and Turner, L. H. (1961). The effect of pH on the survival of leptospires in water. Bull. World Health Organ. 24, 35–43.
Smith, D. J., and Self, H. R. (1955). Observations on the survival of Leptospira australis A in soil and water. J. Hyg. 53, 436–444. doi: 10.1017/S0022172400000942
Soupé-Gilbert, M. E., Bierque, E., Geroult, S., Teurlai, M., and Goarant, C. (2017). Continuous excretion of Leptospira borgpetersenii Ballum in mice assessed by viability-quantitative PCR. Am. J. Trop. Med. Hyg. 97, 1088–1093. doi: 10.4269/ajtmh.17-0114
Stalheim, O. H. (1966). Leptospiral selection, growth, and virulence in synthetic medium. J. Bacteriol. 92, 946–951. doi: 10.1128/jb.92.4.946-951.1966
Sterling, C. R., and Thiermann, A. B. (1981). Urban rats as chronic carriers of leptospirosis: an ultrastructural investigation. Vet. Pathol. 18, 628–637. doi: 10.1177/030098588101800508
Stone, N. E., Hall, C. M., Ortiz, M., Hutton, S., Santana-Propper, E., Celona, K. R., et al. (2022). Diverse lineages of pathogenic Leptospira species are widespread in the environment in Puerto Rico, USA. PLoS Negl. Trop. Dis. 16, e0009959. doi: 10.1371/journal.pntd.0009959
Surdel, M. C., Anderson, P. N., Hahn, B. L., and Coburn, J. (2022). Hematogenous dissemination of pathogenic and non-pathogenic Leptospira in a short-term murine model of infection. Front. Cell. Infect. Microbiol. 12, 917962. doi: 10.3389/fcimb.2022.917962
Sykes, J. E., Gamage, C. D., Haake, D. A., and Nally, J. E. (2022). Understanding leptospirosis: application of state-of-the-art molecular typing tools with a One Health lens. Am. J. Vet. Res. 83, ajvr.22.06.0104. doi: 10.2460/ajvr.22.06.0104
Takeuchi, O., and Akira, S. (2010). Pattern recognition receptors and inflammation. Cell 140, 805–820. doi: 10.1016/j.cell.2010.01.022
Thibeaux, R., Geroult, S., Benezech, C., Chabaud, S., Soupé-Gilbert, M. E., Girault, D., et al. (2017). Seeking the environmental source of Leptospirosis reveals durable bacterial viability in river soils. PLoS Negl. Trop. Dis. 11, e0005414. doi: 10.1371/journal.pntd.0005414
Thibeaux, R., Girault, D., Bierque, E., Soupé-Gilbert, M. E., Rettinger, A., Douyere, A., et al. (2018). Biodiversity of environmental Leptospira: improving identification and revisiting the diagnosis. Front. Microbiol. 9, 816. doi: 10.3389/fmicb.2018.00816
Thibeaux, R., Soupé-Gilbert, M. E., Kainiu, M., Girault, D., Bierque, E., Fernandes, J., et al. (2020). The zoonotic pathogen Leptospira interrogans mitigates environmental stress through cyclic-di-GMP-controlled biofilm production. NPJ Biofilms Microbiomes 6, 24. doi: 10.1038/s41522-020-0134-1
Togami, E., Kama, M., Goarant, C., Craig, S. B., Lau, C., Ritter, J. M., et al. (2018). A large leptospirosis outbreak following successive severe floods in Fiji, 2012. Am. J. Trop. Med. Hyg. 99, 849–851. doi: 10.4269/ajtmh.18-0335
Toyofuku, M., Inaba, T., Kiyokawa, T., Obana, N., Yawata, Y., Nomura, N., et al. (2016). Environmental factors that shape biofilm formation. Biosci. Biotechnol. Biochem. 80, 7–12. doi: 10.1080/09168451.2015.1058701
Trevejo, R. T., Rigau-Perez, J. G., Ashford, D. A., McClure, E. M., Jarquin-Gonzalez, C., Amador, J. J., et al. (1998). Epidemic leptospirosis associated with pulmonary hemorrhage-Nicaragua, 1995. J. Infect. Dis. 178, 1457–1463. doi: 10.1086/314424
Valentini, M., and Filloux, A. (2016). Biofilms and cyclic di-GMP (c-di-GMP) signaling: lessons from Pseudomonas aeruginosa and other bacteria. J. Biol. Chem. 291, 12547–12555. doi: 10.1074/jbc.R115.711507
Vasconcelos, L., Aburjaile, F., Andrade, L., Cancio, A. F., Seyffert, N., Aguiar, E., et al. (2023). Genomic insights into the c-di-GMP signaling and biofilm development in the saprophytic spirochete Leptospira biflexa. Arch. Microbiol. 205, 180. doi: 10.1007/s00203-023-03519-7
Vernel-Pauillac, F., Murray, G. L., Adler, B., Boneca, I. G., and Werts, C. (2021). Anti-Leptospira immunoglobulin profiling in mice reveals strain specific IgG and persistent IgM responses associated with virulence and renal colonization. PLoS Negl. Trop. Dis. 15, e0008970. doi: 10.1371/journal.pntd.0008970
Vernel-Pauillac, F., and Werts, C. (2018). Recent findings related to immune responses against leptospirosis and novel strategies to prevent infection. Microbes Infect. 20, 578–588. doi: 10.1016/j.micinf.2018.02.001
Villanueva, S. Y., Saito, M., Tsutsumi, Y., Segawa, T., Baterna, R. A., Chakraborty, A., et al. (2014). High virulence in hamsters of four dominantly prevailing Leptospira serovars isolated from rats in the Philippines. Microbiology 160(Pt 2), 418–428. doi: 10.1099/mic.0.072439-0
Vincent, A. T., Schiettekatte, O., Goarant, C., Neela, V. K., Bernet, E., Thibeaux, R., et al. (2019). Revisiting the taxonomy and evolution of pathogenicity of the genus Leptospira through the prism of genomics. PLoS Negl. Trop. Dis. 13, e0007270. doi: 10.1371/journal.pntd.0007270
Vinod Kumar, K., Lall, C., Raj, R. V., and Vijayachari, P. (2019). Coaggregation and biofilm formation of Leptospira with Staphylococcus aureus. Microbiol. Immunol. 63, 147–150. doi: 10.1111/1348-0421.12679
Vinod Kumar, K., Lall, C., Vimal Raj, R., Vedhagiri, K., and Vijayachari, P. (2016). Molecular detection of pathogenic leptospiral protein encoding gene (lipL32) in environmental aquatic biofilms. Lett. Appl. Microbiol. 62, 311–315. doi: 10.1111/lam.12533
Wang, J., Zhang, W., Jin, Z., Ding, Y., Zhang, S., Wu, D., et al. (2022). A lethal model of Leptospira infection in hamster nasal mucosa. PLoS Negl. Trop. Dis. 16, e0010191. doi: 10.1371/journal.pntd.0010191
Weinberger, D., Baroux, N., Grangeon, J.-P., Ko, A. I., and Goarant, C. (2014). El Niño Southern Oscillation and leptospirosis outbreaks in New Caledonia. PLoS Negl. Trop. Dis. 8, e2798. doi: 10.1371/journal.pntd.0002798
Wichapeng, S., Chadsuthi, S., and Modchang, C. (2021). Impact of rainfall on the transmission of leptospirosis in Si Sa Ket, Thailand. J. Phys. Conf. Ser. 1719, 012024. doi: 10.1088/1742-6596/1719/1/012024
Witchell, T. D., Eshghi, A., Nally, J. E., Hof, R., Boulanger, M. J., Wunder, E. A. Jr., et al. (2014). Post-translational modification of LipL32 during Leptospira interrogans infection. PLoS Negl. Trop. Dis. 8, e3280. doi: 10.1371/journal.pntd.0003280
Wunder, E. A. Jr., Figueira, C. P., Santos, G. R., Lourdault, K., Matthias, M. A., Vinetz, J. M., et al. (2016). Real-time PCR reveals rapid dissemination of Leptospira interrogans after intraperitoneal and conjunctival inoculation of hamsters. Infect. Immun. 84, 2105–2115. doi: 10.1128/IAI.00094-16
Xiao, G., Kong, L., Che, R., Yi, Y., Zhang, Q., Yan, J., et al. (2018). Identification and Characterization of c-di-GMP metabolic enzymes of Leptospira interrogans and c-di-GMP fluctuations after thermal shift and infection. Front. Microbiol. 9, 764. doi: 10.3389/fmicb.2018.00764
Yamaguchi, T., Higa, N., Okura, N., Matsumoto, A., Hermawan, I., Yamashiro, T., et al. (2018). Characterizing interactions of Leptospira interrogans with proximal renal tubule epithelial cells. BMC Microbiol. 18, 64. doi: 10.1186/s12866-018-1206-8
Yanagihara, Y., Villanueva, S. Y. A. M., Nomura, N., Ohno, M., Sekiya, T., Handabile, C., et al. (2022). Leptospira is an environmental bacterium that grows in waterlogged soil. Microbiol. Spectr. 10, e02157-121. doi: 10.1128/spectrum.02157-21
Zhang, Q., Zhang, Y., Zhong, Y., Ma, J., Peng, N., Cao, X., et al. (2011). Leptospira interrogans encodes an ROK family glucokinase involved in a cryptic glucose utilization pathway. Acta Biochim. Biophys. Sin. 43, 618–629. doi: 10.1093/abbs/gmr049
Zhang, Y., Lou, X. L., Yang, H. L., Guo, X. K., Zhang, X. Y., He, P., et al. (2012). Establishment of a leptospirosis model in guinea pigs using an epicutaneous inoculations route. BMC Infect. Dis. 12, 20. doi: 10.1186/1471-2334-12-20
Keywords: epidemiological cycle, environmental survival, environmental contamination, biofilm, c-di-GMP, mammal host infection
Citation: Davignon G, Cagliero J, Guentas L, Bierque E, Genthon P, Gunkel-Grillon P, Juillot F, Kainiu M, Laporte-Magoni C, Picardeau M, Selmaoui-Folcher N, Soupé-Gilbert M-E, Tramier C, Vilanova J, Wijesuriya K, Thibeaux R and Goarant C (2023) Leptospirosis: toward a better understanding of the environmental lifestyle of Leptospira. Front. Water 5:1195094. doi: 10.3389/frwa.2023.1195094
Received: 28 March 2023; Accepted: 10 July 2023;
Published: 27 July 2023.
Edited by:
Kovashnee Naidoo, National Health Laboratory Service (NHLS), South AfricaReviewed by:
Claudia Munoz-Zanzi, University of Minnesota, United StatesMohd Hatta Abdul Mutalip, Ministry of Health, Malaysia
Sharon Yvette Angelina Manalo Villanueva, University of the Philippines Manila, Philippines
Nattachai Srisawat, Chulalongkorn University, Thailand
Copyright © 2023 Davignon, Cagliero, Guentas, Bierque, Genthon, Gunkel-Grillon, Juillot, Kainiu, Laporte-Magoni, Picardeau, Selmaoui-Folcher, Soupé-Gilbert, Tramier, Vilanova, Wijesuriya, Thibeaux and Goarant. This is an open-access article distributed under the terms of the Creative Commons Attribution License (CC BY). The use, distribution or reproduction in other forums is permitted, provided the original author(s) and the copyright owner(s) are credited and that the original publication in this journal is cited, in accordance with accepted academic practice. No use, distribution or reproduction is permitted which does not comply with these terms.
*Correspondence: Roman Thibeaux, thibeaux@pasteur.nc