- ICMR-National Institute of Malaria Research, New Delhi, India
The long term in vitro culture of Plasmodium falciparum was successfully established by Trager and Jensen in 1976; however it largely remains unachieved for P. vivax. The major obstacle associated with Plasmodium vivax in vitro culture is its predilection for invading younger reticulocytes and the complex remodelling of invaded reticulocytes. There are many factors under exploration for this predilection and host–parasite interactions between merozoites and invaded reticulocytes. These include various factors related to parasite, host and environment such as compromised reticulocyte osmotic stability after invasion, abundance of iron in the reticulocytes which makes them favourable for P. vivax growth and propagation and role of a hypoxic environment in P. vivax in vitro growth. P. vivax blood stage transfection represents another major hurdle towards understanding this parasite’s complex biology. Efforts in making this parasite amenable for molecular investigation by genetic modification are limited. Newer approaches in sustaining a longer in vitro culture and thereby help advancing transfection technologies in P. vivax are urgently needed that can be explored to understand the unique biology of this parasite.
Background
Plasmodium vivax is the most widespread Plasmodium species, causing the second highest mortality and morbidity from malaria after Plasmodium falciparum. According to the World Health Organization (2021), although the proportion of estimated P. vivax cases in the world went down from 8% in 2000 to 2% in 2020, P. vivax contributes an estimated 36% and 30% of all malaria cases in WHO South East Asia and Western Pacific region, respectively. It remains the dominant species (>50% of local cases) in Bhutan, Korea, Myanmar, Nepal and Thailand in 2020 (World Health Organization, 2021). Plasmodium vivax grabbed clinical attention only since last decade due to reports presenting P. vivax associated severe malaria and deaths (Kochar et al., 2005, 2009; Baird, 2007; Price et al., 2007; Anstey et al., 2009; Alexandre et al., 2010; Costa et al., 2011; Douglas et al., 2013, 2014; Patriani et al., 2019). Plasmodium vivax is also thought to be a major barrier in malaria elimination and eradication, mostly because of its chronic and relapsing symptoms. Despite enormous clinical and public health importance, P. vivax has not been able to take hold of research attention it deserves mainly because of the two major bottlenecks in understanding the clinical and basic biology of the parasite-lack of long-term in vitro culture of its blood stages and sub-optimal development of transfection technologies for its genetic manipulation. Therefore, this review focuses on latest updates on these two hurdles and suggests potential ways to circumvent them.
Contextual plasmodium vivax biology
The life cycle and biology of Plasmodium vivax (Figure 1) is unlike other Plasmodium species. Its distinguishing features involves: (i) development of sporozoite into a dormant form in hepatocytes called hypnozoites (Cogswell, 1992), (ii) P. vivax merozoites’ predilection towards reticulocytes (Field and Shute, 1956) unlike other species of Plasmodium and this possibly results in significantly lower parasitemia, (iii) Presence of spherical shaped gametocytes in the peripheral blood before the appearance of clinical symptoms (Boyd and Kitchen, 1937), (iv) Presence of all blood stage developmental forms (ring, trophozoite, schizont, and gametocytes) in the peripheral blood. However, the mature stages, i.e., trophozoite and schizont are less common in peripheral blood than immature stages (Rudolf and Ramsay, 1927; Field and Shute, 1956; Lopes et al., 2014).
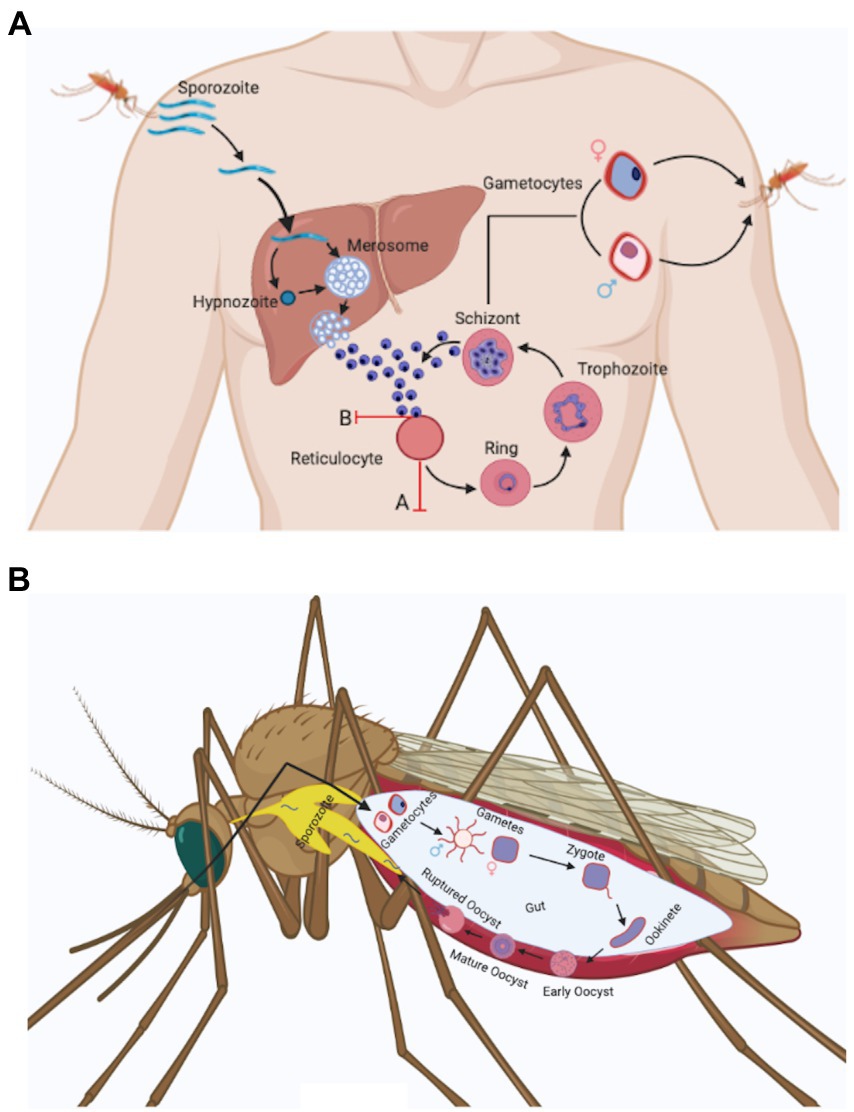
Figure 1. Plasmodium vivax life cycle. (a) Anopheles spp. mosquito inoculates Plasmodium sporozoites into the dermis of human skin that migrate to blood stream and finally invade liver. In the liver, Plasmodium sporozoites differentiate either into schizonts or into a dormant form called as hypnozoites that can activate after months or years and release merozoites. Plasmodium vivax hepatic merozoites invade reticulocytes in the bloodstream and start the formation of caveola-like complexes that helps in the completion of blood schizogony. Some of the asexual parasites undergo gametocytogenesis and form rounded gametocytes (after 4 days of infection) that are sucked up along with blood meal by another Anopheles mosquito (Bourgard et al., 2018). Two major obstacles associated with Plasmodium vivax in vitro culture are shown in the figure with red marks. These include: (A) Reticulocyte maturation: Reticulocytes mature rapidly in vitro condition (Malleret et al., 2015). (B) Loss of invasion: Plasmodium vivax merozoites lose their ability to invade uninfected reticulocytes in vitro after a few cycles (Bermudez et al., 2018). (b) Male and female gametocytes taken up by the mosquito differentiate into male and female gametes and fuse to form zygote in the mosquito mid gut. The zygote differentiates into motile ookinetes which cross the midgut epithelium and divide further to form oocyst. The oocyst ruptures to release hundreds of sporozoites that traverse to salivary glands and can be transmitted to a new host with a fresh mosquito bite (Mueller et al., 2009).
After the bite of an infected female Anopheles mosquitoes, sporozoites move from skin to liver in <30 min, infect hepatocytes and matures to form an actively dividing hepatic schizont or an inactive hypnozoite (Figure 1; Krotoski, 1985). The hypnozoite may remain in quiescent state for months or years and instigates clinical relapse(s) after entering the bloodstream. The cause behind the activation of hypnozoites is not explored, though parasitic and bacterial infection could be a probable factor (Shanks and White, 2013). In coendemic regions where both P. vivax and P. falciparum exist, a high risk of P. vivax parasitaemia was observed after P. falciparum treatment (Douglas et al., 2011; Commons et al., 2019). A meta-analysis performed by Commons et al. demonstrated that after 63 days of treatment of P. falciparum infection the risk of P. vivax recurrence was 24% and it represented almost 70% of all parasitological recurrences. However, the mechanism behind the increased risk of P. vivax parasitaemia after treatment of P. falciparum infection remains unclear, it has been hypothesised to be due to a possible relapse of P. vivax hypnozoites (Hossain et al., 2020).
Another unique feature of P. vivax is its predilection for reticulocytes as host cells (Kitchen, 1938). Reticulocytes represent 1%–2% of total red blood cells and this is the probable reason of lower parasitemia in P. vivax infected patients. Reticulocytes are larger than erythrocytes (Riley et al., 2001) therefore P. vivax infected cells appear larger as compared to uninfected/P. falciparum-infected erythrocytes present in peripheral blood smear. P. vivax during its development period inside the host cells produces specific proteins which makes cleft like structure in the host cell membrane and caveolae-vesicle complexes which looks like small dots in Giemsa–stained smear called as Schuffner’s dots (Aikawa et al., 1975; Mueller et al., 2009; Akinyi et al., 2012; Gunalan et al., 2020). The biological role of these dots remains largely unexplored but these dots are inconspicuous during P. vivax cultures (Gunalan et al., 2020).
One of the unique identifying feature of P. vivax is, the amoeboid shape (cytoplasm having finger like projections) of red blood cells infected with this parasite (Suwanarusk et al., 2004). Suwanarusk et al. (2004) experimentally showed that the P. vivax infected red blood cells (Pv-iRBCs) increase in deformability so that it can pass through the sinusoid of the spleen easily. The increased deformability of Pv-iRBCs may represent a way to avoid its clearance from spleen (Suwanarusk et al., 2004; Handayani et al., 2009). Further, this may lead to the passage of all parasite stages in the peripheral blood (Suwanarusk et al., 2004). Presence of all stages in peripheral blood and lack of the adhesive knobs in P. vivax suggestedlack of cytoadhesion and sequestration in deep capillaries. However, presence or absence of sequestration and cytoadhesion in P. vivax remains a dogma as few reports suggested cytoadhesion to spleen (del Portillo et al., 2004) and lungs (Anstey et al., 2007). In Field et al. (1963) proposed sequestration of P. vivax, as disappearance of schizonts from the peripheral blood of P. vivax infected patient was observed (Field et al., 1963). Another recent report, showed absence or very low presence of schizonts in peripheral blood (Lopes et al., 2014). In addition, accumulation of schizont and gametocytes in bone marrow was also observed (Baro et al., 2017). The extracellular vesicles (EVs) present in the plasma of P. vivax infected patients contain parasite protein that facilitate the adhesion of infected reticulocytes to human spleen fibroblasts (hSFs). Human spleen fibroblast uptakes the P. vivax infected EVs that induces the expression of ICAM1 after the binding of transcription factor NF-kB (NF-kB translocates from cytosol to nucleus). Increased expression of ICAM-1 on hSFs membrane facilitates reticulocytes sequestration where parasites cytoadhere and multiply in spleen microvasculature (Toda et al., 2020). This report further supports the hypothesis of P. vivax cytoadherence and sequestration.
Nevertheless, this Plasmodium species contains circular gametocytes similar to most of the Plasmodium genus except Plasmodium falciparum and Plasmodium reichenowi (Mueller et al., 2009) which contain elongated gametocytes. Another characteristic that has clinical significance is that the P. vivax gametocytes appear early (after 4 days of infection) and can be observed in the peripheral blood before the onset of clinical manifestation (Mueller et al., 2009). Report suggests gametocyte production may start with the first generation of merozoites (McKenzie et al., 2002). However, it remains unclear when the sexual commitment does start.
Plasmodium vivax in vitro culture
Continuous long-term in vitro culture (Plasmodium falciparum, Plasmodium knowlesi, and Plasmodium cynomolgi) has proved to be a crucial tool for understanding the parasite’s life cycle and invasion process in detail (Trager and Jensen, 1976; Kocken et al., 2002; Grüring et al., 2014; Chua et al., 2019). However, such a system for long-term in vitro culture does not exist for Plasmodium vivax. Developing a method that allows the continuous growth and propagation of Plasmodium vivax would provide us new research opportunities to explore the molecular details of Plasmodium vivax malaria. The limitations and research breaches of P. vivax blood stage culture have been discussed here in detail. In addition, alternate research avenues being developed and explored by vivax malariologists, have also been discussed.
The first successful P. vivax in vitro culture was reported in 1912 (Bass and Johns, 1912). However, the detailed protocol for propagating this parasite was established only by the mid of 1970s (Trager and Jensen, 1976). Since then, several modifications have been attempted to improve the culture conditions by modifying the culture media, parasite origin and reticulocyte origin as reviewed recently in detail by others (Bermudez et al., 2018; Thomson-Luque et al., 2019). A timeline representing the major developments towards P. vivax culture optimization has been shown in Figure 2. In a major development, P. vivax culture was maintained continuously for 233 days (0.1% parasitemia) using new world monkey (Saimiri boliviensis) blood along with DMEM (Dulbecco’s Modified Eagle Medium), L-glutamine, HEPES (4-(2-hydroxyethyl)-1-piperazineethanesulfonic acid) and hypoxanthine (Mehlotra et al., 2017). This study leads to the belief that long term culture of P. vivax culture is not impossible anymore. However, further validation is required with human peripheral blood.
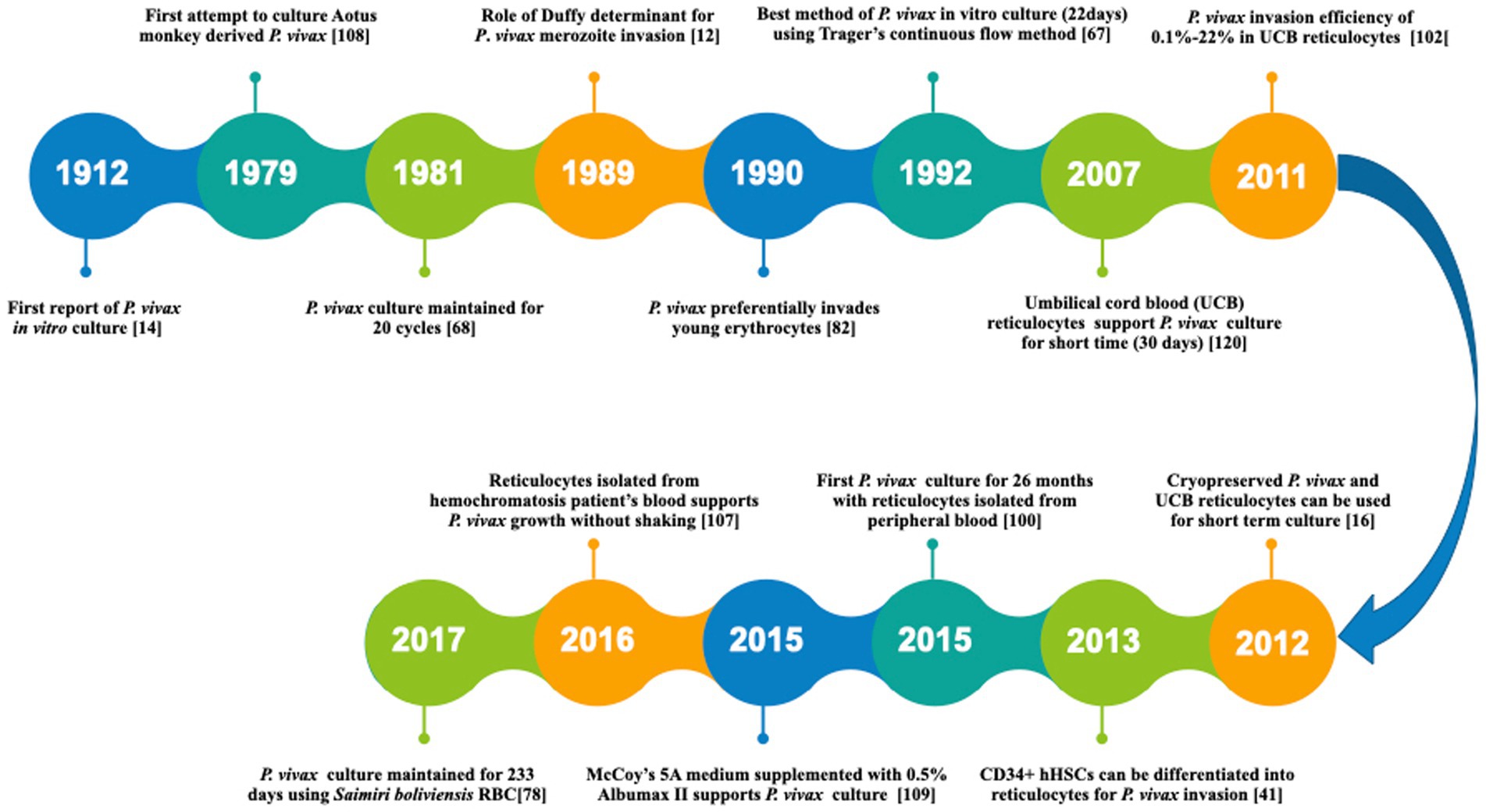
Figure 2. A timeline representing the major developments towards P. vivax in vitro culture. [14]: Bass and Jones (1912) [108]: Siddiqui (1979) [68]: Larrouy et al. (1981) [12]: Barnwell et al. (1989) [82]: Mons (1990) [67]: Lanners (1992) [120]: Udomsangpetch et al. (2007) [102]: Russell et al. (2011) [16]: Borlon et al. (2012) [41]: Fernandez-Becerra et al. (2013) [100]: Roobsoong et al. (2015) [109]: Singh et al. (2015) [107]: Shaw-Saliba et al. (2016) [78]: Mehlotra et al. (2017).
Despite continuous efforts to culture P. vivax, it remains difficult primarily because of the progressive loss of parasite’s invasion efficiency, i.e., the percentage of infective merozoites that are able to invade and establish infection in reticulocytes (Thomson-Luque et al., 2017; Bermudez et al., 2018; Gunalan et al., 2020). However, the biological basis of the loss of parasites’ invasion efficiency is far from clear. A thorough analysis is needed to be done considering host, parasite and environmental factors. Here, an attempt has been made to understand all the three factors in detail.
Host factors
Plasmodium vivax requires host reticulocytes for its growth and propagation (Bass and Johns, 1912; Mons, 1990). Reticulocytes are precursor red blood cells, slightly larger (10–15 mm) than the mature red cells (6–8 mm) and having reticular network of residual RNA. Heilmeyer and Westhauser observed diverse nature of reticulocytes in supravital stained blood smear and classified them in four classes (I–IV). A very young reticulocyte without nucleus having dense coherent mass of RNA along with other organelles is called as Heilmeyer class I (HCI) reticulocyte. Further mature reticulocytes having reticular network of RNA instead of original dense mass are called as HCII reticulocyte. HCIII reticulocytes have lesser amount of dispersed RNA. The most mature reticulocytes having dispersed remnants of RNA are called as HCIV reticulocytes (Heilmeyer and Westhäuser, 1932). Whereas HCI and HCII early stage reticulocytes are present in bone marrow, HCIII reticulocytes are mostly found the in bone marrow and HCIV are the most common form of reticulocytes present in the peripheral blood (Seip, 1953). Early stage reticulocytes are large, rigid, and irregular in shape but mature reticulocytes become smaller, and biconcave in shape (Malleret et al., 2013). Reticulocytes represent only 1%–2% of circulating human red blood cells and have a short life span of ~4 days:3 days in bone marrow and 24 h in circulation (Kaufman, 2017).
The maturation of reticulocytes is indicated by decreased expression of transferrin receptor (CD71) (Kono et al., 2009; Malleret et al., 2013). The majority of immature CD71+ reticulocytes (HC0, I, II, and III) are restricted to the bone marrow and remain more invasive to P. vivax as compared to the CD71− cells (HCIV reticulocytes or erythrocytes) and minor population of CD71+ reticulocytes present in the peripheral blood (Malleret et al., 2015). CD71 has been shown to be a critical reticulocyte receptor for invasion by P. vivax through the parasite ligand, PvRBP2b (Gruszczyk et al., 2018) along with the P. vivax Duffy-binding protein/Duffy antigen receptor for chemokines channel (Kanjee et al., 2021). The preferential invasion in CD71+reticulocytes may be because immature reticulocytes are rich in iron and other metabolites (Thomson-Luque and Bautista, 2021) which are required for parasite maturation. Immature reticulocytes synthesise haemoglobin and ~20%–30% of total haemoglobin is synthesised at this stage of the red blood cells (Riley et al., 2001). Nevertheless, Malleret et al. revealed that P. vivax induces rapid maturation of reticulocytes ex vivo, may be to protect itself from the continuous deformations of immature reticulocytes (Malleret et al., 2015). Later, Lim et al. confirmed that red blood cell maturation does not happen so rapidly in vivo as early stage parasites are found to be present always in reticulocytes (Lim et al., 2016). However, understanding the specific crosstalk between maturing P. vivax and maturing reticulocytes may have impact on long term in vitro cultivation of P. vivax.
CD71 (transferrin receptor1) being an iron importer protein, brings transferrin bound iron inside the reticulocytes (Jandl et al., 1959). Interestingly, reticulocytes isolated from haemochromatosis patients represented 14%–17% of total red blood cells compared to 6.9%–7.9% from umbilical cord reticulocytes (Udomsangpetch et al., 2007). Haemochromatosis is an inherited disorder characterised by excess iron accumulation in tissues and organs (Andrews et al., 1999) andphlebotomy is an effective treatment of hemochromatosis as it removes excess iron present in blood. Post-phlebotomy, haemoglobin concentration and serum iron level decreases and bone-marrow becomes highly active leading to increased reticulocytosis to compensate red blood cell loss and a slower reticulocyte maturation rates (Conrad et al., 1962; Gildersleeve et al., 1985; Feeney et al., 2005). Further, there are reports showing severe anaemia in case of P. vivax infection despite having low parasitic burden (Mohapatra et al., 2002; Collins et al., 2003; Kenangalem et al., 2016; Punnath et al., 2020). One of the reasons could be that P. vivax may require more iron for its growth and survival which results in host iron scarcity and ultimately anaemia. In one of the most successful attempts to culture P. vivax till date, Golenda et al. used haemochromatosis patient’s reticulocytes and observed almost 2-fold increase in parasitemia after each cycle which was higher compared to other methods (Panichakul et al., 2007; Noulin et al., 2012, 2014). According to a recent report by Shaw-Saliba et al., P. vivax showed significant preference for reticulocytes isolated from fresh haemochromatosis patients’ blood. They achieved a maximum of 1.8% parasitemia using McCoy’s 5A media supplemented with 20% AB+ heat inactivated serum, 6% haematocrit in a candle jar (~18% oxygen; Shaw-Saliba et al., 2016). Noteworthy, Golenda et al. achieved 5-fold increase in the number of reticulocytes after centrifugation steps whereas Shaw-Saliba et al. could obtain a maximum of 3-fold increase in reticulocyte from starting hemochromatosis blood. It could be because of differences in reticulocyte count which may be due to different treatment procedure, different number of bleeds and phlebotomy of hemochrombtosis patients’ (Shaw-Saliba et al., 2016). Altogether it seems that reticulocytes isolated from haemochromatosis patient blood remains more invasive to P. vivax infection compared to other blood sources, possibly because of the abundance of iron and more number of CD71+ reticulocytes in haemochromatosis blood (Conrad et al., 1962; Gildersleeve et al., 1985; Feeney et al., 2005).
A recent report by Gunalan et al. suggested that oxidative stress due to higher (as compared to that in bone marrow) ambient O2 during various culture conditions/steps could have negative effect on P. vivax growth. Further, reticulocytes’ high glucose-6-phosphate dehydrogenase (G6PD) content (that reduces as RBCs mature) counteracts this oxidative stress and hence offer a congenial micro-environment for P. vivax growth (Luzzatto, 2006; Wilson et al., 2016; Gunalan et al., 2020). However, supplementation of antioxidants like glutathione, ascorbic acid, selenium, pyruvate, and lipoic acid does not improve P. vivax growth. It is further suggested that a combination of antioxidants along with other culture-condition modifications, including culture in hypoxia-mimicking conditions and use of more membrane-permeable antioxidant compounds, might help in relieving the oxidative stress present inside the reticulocytes (Gunalan et al., 2020). It is noteworthy that iron (Fe+2) also acts as pro-oxidant and can induce cellular oxidative stress (Puntarulo, 2005; Colpo et al., 2008). It would be interesting to examine if iron chelators can be used to mitigate the cellular oxidative stress that was induced by iron (Fe+2). Adding to the list of antioxidants that were described by Gunalan et al., deferoxamine, deferiprone and deferasirox may also be explored.
Clark et al. examined the structural integrity of Plasmodium vivax infected reticulocytes using a flowcytometry-based assay that measured the osmotic stability of reticulocytes. It was observed that immature CD71+ reticulocytes were osmotically most stable amongst other mature stages of CD71− reticulocytes and normocytes. P. vivax infected reticulocytes were less stable compared to uninfected CD71+ reticulocytes, suggesting that P. vivax infection compromises the reticulocyte stability. In addition, it was observed that P. vivax-infected reticulocytes were more instability as compared P. falciparum-infected normocytes. Interestingly, reticulocytes acquired after in vitro differentiation of stem cells have been shown to be less stable compared to ex vivo reticulocytes (Clark et al., 2021). This could be one of the reasons behind the observation that P. vivax better propagates in ex vivo culture. It thus implies that the reagents that can increase the osmotic stability of reticulocytes in vitro, would have impact on long term culture of Plasmodium vivax and, to cite an example, cholesterol has been shown to increase the osmotic stability of reticulocytes present in in vitro culture (Clark et al., 2021).
Rapid maturation of reticulocytes also turns out to be a restrictive factor when using these cells for assays which take a longer time to reach to the assay endpoint. Borlon et al. analysed the probability of cryopreserving reticulocytes which were obtained from umbilical cord blood. Reticulocytes were isolated as per the earlier established protocol (Russell et al., 2011). Subsequently, cells were homogenised in 20% glycerol and preserved in liquid nitrogen. It was shown that liquid nitrogen preservation did not affect reticulocytes stability and viability as they matured normally and supported parasite invasion (Borlon et al., 2012). Further, Noulin et al. showed that erythrocytes obtained from haematopoietic stem cells could be cryopreserved for 1 year without disturbing the receptors present on the cell membrane. Two different media containing different preservative agents were used for reticulocytes cryopreservation and both were successful. The first consisted of 28% glycerol, 3% sorbitol and 0.9% sodium chloride, whereas the other contained 10% dimethyl sulfoxide (DMSO) and 40% fetal calf serum (Noulin et al., 2012).
Parasite factors
Despite the continuous supply of fresh reticulocytes, P. vivax culture remains challenging due to a variety of parasite-associated variables, including inefficient invasion after few rounds of asexual intra-erythrocytic cycles, lack of maturation and egress. The incompetent parasite invasion to reticulocytes under in vitro condition remains one of the biggest hurdles (Thomson-Luque et al., 2017). Thomson-Luque has enlisted some of the problems observed during P. vivax in vitro culture: pyknotic rings, lack of Schuffner dots, delayed trophozoite formation, presence of gametocytes instead of blood stages, appearance of crisis forms of parasites (broken pieces of parasites or nutrient deficient unhealthy parasites; Thomson-Luque et al., 2017). Nonetheless, decrease in parasitemia was observed mostly at the trophozoite to schizont transition state. The reason behind this enigma remains unexplored. However, lack of a favourable environment could be one of the factors.
Plasmodium vivax isolates are genetically divergent in nature as each isolate having different level of reticulocyte preference. A study by Lim et al. using Indian P. vivax isolates showed that some of the P. vivax strains have less predilection towards reticulocytes in contrast to the reticulocyte restricted nature of the species, in general. Strains which have preference for reticulocytes, had more number of schizonts than gametocytes, suggested invasion to young reticulocyte helps in schizont development (Lim et al., 2016). In another study, Russell et al. examined invasion efficiency of the parasite using 85 clinical isolates of P. vivax from Thailand-Myanmar border. A high level of variation was observed in invasion efficiencies amongst all the P. vivax clinical isolates. However, invasion efficiency remains constant when a specific isolate was incubated with reticulocytes isolated from different ABO blood group donors. Altogether, it was observed that ~86% of the total variance in invasion efficiency was dependent on the parasite isolate type. Host blood group accounted for only 0.17% of total variation in invasion efficiency (Russell et al., 2011).
Both of the above mentioned studies indicate that each P. vivax human isolates has its own characteristics and this creates variations in invasion. Further, the unique characteristics of each isolate could be attributed to hitherto unknown genetic factors. These genetic determinants might regulate individual parasite isolate at transcriptomic, genomic or epigenomic levels. In future, the determinant that regulates the reticulocytes invasion is expected to have impact on long term blood stage culture of P. vivax.
Regular parasite-based invasion assays measure the average parasitemia from a group of parasites, assuming the average parasitemia is representative of each parasite’s invasion. However, in doing so a particular subpopulation of parasite may not be counted whose behaviour is different from rest of the parasites. Therefore, single parasite culture should be performed as standardised by others (Miao and Cui, 2011) and its growth pattern should be monitored. Fluorescence activated cell sorting (FACS) based single parasite isolation from complex parasite population could provide insights on the genetic variation at individual parasite level.
Environmental factors
The third most important factor involved with P. vivax in vitro culture is the culturing medium (the environment present around the parasite). The detail regarding the culture media formulation for P. vivax in vitro culture, has already been reviewed by others in detail (Bermudez et al., 2018; Thomson-Luque et al., 2019). Therefore, it has not been discussed here. Moreover, McCoy’s 5A medium with human serum is the most commonly used formulation for P. vivax propagation in in vitro condition. However, McCoy’s 5A formulation has been reported to reduce P. vivax parasites’ viability after 48 h of intraerythrocytic cycle (Borlon et al., 2012). Recent observation by Rengel et al. show that Iscove’s modified Dulbecco’s medium (IMDM) supports parasites’ intraerythrocytic growth better compared to other formulations (Rangel et al., 2018). However, no significant difference was observed in transcriptomic signature distinguishing parasites grown in IMDM versus other media. Nonetheless, Plasmodium vivax parasites’ density does decline over time even with IMDM and it represents a major obstacle to sustained in vitro culture of this parasite.
FACS-assisted single cell culture of P. vivax infected reticulocytes can be performed in order to explore the inhibitory effect of one parasite on the growth and survival of neighbouring parasites and subsequently parasite invasion, maturation, egress can be monitored. Further, several reports suggest that each P. vivax isolates has its unique behaviour in in vitro condition. Isolate specific metabolomics study may help in understanding the differences in metabolic pathways operational in in vitro condition. The metabolomics exploration study would aid in optimization of culture media and growth factors required for each isolate. Nevertheless, Rengel et al. attempted similar study, in which 1000 FACS-purified parasites from viable cryopreserved patient isolates were cultured in different media formulations and transcriptome analysis was performed at various parasite development stages. Further, it was observed that culture media has not much effect on parasites’ transcriptional profile (Rangel et al., 2020). The lack of parasites’ transcriptional response in different culture media might indicate the altered role of host cells (red blood cells) itself. However, more studies required in order to explore the role of different media on Parasites’ survival. In addition, single-cell transcriptomics approaches would help further in characterising the variation between the parasites within an isolate.
Altogether, the parasite, environment and host factors appear to the determining factors for Plasmodium vivax in vitro culture, either singly or in combination. This includes the role of parasite genes and gene expression in maintaining parasite invasion and parasite biomass during in vitro cultures. Further, Plasmodium vivax parasites lack molecular and genetic determinants required for drug selection and identification of biomarkers. Development of P. vivax transgenic parasites has suffered a stback from lack of an in vitro culture system together with low transfection efficiency and lack of selection markers. Despite these challenges, few attempts have been made in order edit this parasite at molecular level.
Plasmodium vivax blood stage transfection
Despite the development of emerging methodologies for genome editing, the genetic manipulation of Plasmodium vivax still remains poorly attempted. The lack of efforts to genetically manipulate this parasite is mostly attributed to the absence of long term in vitro culture and a poor availability of molecular tools to genetically manipulate the parasite. This is because stable gene manipulation generally requires a continuous maintenance of 3–4 weeks of asexual propagation and by that time P. vivax may not survive in vitro conditions due to the reasons mentioned above. In case of stable transfection, plasmid DNA initially replicates episomally in the cytoplasm and subsequently during drug selection (for 2–3 weeks), it integrates into the genome by single or double crossover homologous recombination and such successful transformants are further confirmed through Southern blotting (Crabb et al., 1997). In contrast, transient transfection can be performed easily with P. vivax as it requires only 48–96 h of in vitro maintenance after transfection (Crabb et al., 2004). However, a transgenic P. vivax line development and gene function analysis through knockout study requires stable transfection.
Another important factor associated with Plasmodium vivax transfection is the lack of gene regulatory elements required for P. vivax specific plasmid construction. Plasmodium falciparum histidine rich protein3 promoter and calmodulin promoter has been shown to work in P. vivax (Pfahler et al., 2006). Moraes Barros et al. were successful in using P. vivax calmodulin promoter for zinc finger nucleases (ZFN) expression in P. vivax (Moraes Barros et al., 2015). However, several attempts to characterise P. vivax promoter in Plasmodium falciparum blood stage culture remains unsuccessful (Azevedo and del Portillo, 2007). Nevertheless, for the first time P. vivax centromere from chromosome11 (PvCEN) and promoter of heat shock protein 70 (pvhsp70) was shown to be active in P. yoelii (Thawnashom et al., 2019). These regulatory sequences can be utilised for P. vivax specific plasmid construction and gene manipulation in future.
Plasmodium vivax transfection would be a crucial step towards making the parasite more compliant to molecular inquest. Till date, only two independent attempts have been made to transfect P. vivax trophozoites. Pfahler et al. transiently transfected P. vivax blood stage parasites using Luciferase construct (Pfahler et al., 2006). P. vivax SalvadorI strain was used for transfection and parasites were isolated from infected splenectomised Saimiri boliviensis monkeys. The purified P. vivax infected trophozoites were mixed with Luciferase-containing plasmids and electroporated using a Gene Pulser Xcell™ electroporation system (BioRad). The transfected reporter genes were expressed despite being under the control of P. falciparum 5′ and 3′ regulatory elements. This indicated that the regulatory regions are conserved between both the species. In another major development in transfection technology, Morales Barros et al., stably transfected P. vivax Chesson strain using zinc-finger nucleases. A specific mutation (quadruple mutant) was introduced to the gene encoding P. vivax dihydrofolate reductase (pvdhfr) and it was selected using the drug pyrimethamine in Saimiri boliviensis. Blood samples were collected from P. vivax Chesson infected splenectomised Saimiri boliviensis monkey and infected erythrocytes were electroporated ex vivo using ZFN plasmid containing mutant pvdhfr sequence (Moraes Barros et al., 2015). Customised ZFNs can now be used for genetic manipulations in P. vivax. Further, mutant pvdhfr or human dhfr or other selectable markers can be used for selection in P. vivax. In absence of continuous in vitro P. vivax culture system, transfection and selection has been done in expensive nonhuman primates.
Transfection associated variables such as electroporation condition or P. vivax developmental stage to be transfected, has not been optimised due to the limited availability of P. vivax infected red blood cells (Pfahler et al., 2006). However, from the above two transfection study it appears that P. vivax infected blood containing all the developmental stages can be used for transfection unlike other Plasmodium species (Table 1) which prefer a specific stage. Anyway, a stage specific transfection needs to be done in order to explore the transfection efficiency of each developmental stage of P. vivax.
Options to study Plasmodium vivax biology
Reticulocyte producing cell lineage
Reticulocyte availability is the limiting factor in success of P. vivax in vitro culture. Therefore, there is need of a reticulocyte producing cell lineage that can supply uninterrupted, homogenous population of reticulocytes. To date, few attempts have been made to generate immortalised lines of human erythroid cells from haematopoietic stem/progenitor cells (Kurita et al., 2013; Huang et al., 2014), embryonic stem cells (Hirose et al., 2013) or induced pluripotent stem (iPS) cells (Kurita et al., 2013). However, the enucleation rate of iPS cells are less compared to embryonic stem cells. In addition, reticulocytes produced from embryonic stem cells contain fetal haemoglobin that may have adverse effect on in vitro culture. Hematopoetic stem cells isolated from bone marrow or peripheral blood represents a good alternative for reticulocyte line generation. However, bone marrow derived stem cells isolation remain invasive whereas stem cells derived from peripheral blood are easily accessible.
Recently, normal human erythroid cells has been used for making immortalised adult erythroid line (Trakarnsanga et al., 2017). Bristol Erythroid line Adult (BEL-A) is the first human immortalised adult erythroid line derived from adult bone marrow CD34+ cells that generates mature reticulocytes after enucleation. Morphologically and functionally BEL-A resembles that of in vitro cultured reticulocytes (Trakarnsanga et al., 2017). Further, BEL-C and BEL-P erythroid line have been generated from cord blood and peripheral blood CD34+ cells (Daniels et al., 2021). Both, BEL-C and BEL-P has been shown to recapitulate the characteristics of their parental cells in terms of global expression, differentiation profile and overall proteome profile. Moreover, generation of BEL-A, BEL-C and BEL-P lines have open a door to study host–parasite interaction and its aftereffects. Next, the suitability of these lines for P. vivax infection and in vitro culture can be examined.
Humanised mice model
CD71 producing human red blood cells are the limiting factor for P. vivax in vitro culture. Luiza-Batista et al. (2022) attempted to develop a mice model having human erythropoiesis after engraftment of human haematopoietic stem and progenitor cells. This mice model supported de novo P. vivax growth and proliferation after 3-weeks of infection. The mouse strain called as HIS-HEry (Human Immune System-Human Erythrocyte) survived for 1 year and mimicked several key features of P. vivax infected humans including localisation of sexual stages of parasite to bone marrow, gemetocyte formation and transmission to Anopheles mosquitoes despite having low parasitaemia. Though the current model has opened new avenues in understanding Plasmodium vivax biology, due improvements are required, towards low number of circulating human RBCs and low number of sporozoite production after gametocyte transmission (Luiza-Batista et al., 2022). Notably, the low number of peripheral human RBCs attributes to their elimination by murine macrophages (Hu et al., 2011). Further, low number of peripheral RBCs restricts the numbers of gametocytes transmissible to mosquitoes and therefore, the current mice model requires further optimisation.
Primate malaria model – plasmodium cynomolgi
Plasmodium cynomolgi is a relapsing primate malaria parasite and currently it is used as a model system for P. vivax. Both, phylogenetically and phenotypically, P. cynomolgi and P. vivax are quite close to each other (Tachibana et al., 2012). An improved genome sequence assembly of P. cynomolgi revealed 282 similar gene clusters between P. cynomolgi and P. vivax, this further strengthens that they are close related (Pasini et al., 2017) The blood stage culture of P. cynomolgi line derived from Berok strain, was recently developed by Chua et al. (2019). The availability of P. cynomolgiin vitro culture would open new avenues to P. vivax research as it can be utilised for high-throughput drug screening and genetic manipulation which remains unachieved for P. vivax. Successful in vitro propagation of P. cynomolgi Berok K4 line was obtained in six independent laboratories. Mature schizont formed after 46–48 h of infection with eight to 16 merozoites whereas gametocytes observed from day6 onwards and overall gametocytaemia remains low with 0.01%. Noteworthy, P. cynomolgi M and B strains failed several attempt to culture in vitro (Chua et al., 2019).
Plasmodium vivax gene manipulation via Plasmodium knowlesi
Plasmodium vivax remains distinct from P. knowlesi in terms of hypnozoite formation and its preference for reticulocytes. However, the two parasites are phylogenetically closely related and dependent on Duffy binding proteins for host cell invasion (Adams et al., 1990; King et al., 2011). In absence of long-term in vitro culture, the P. vivax research is mostly constrained to recombinant protein assays, ex vivo studies, primate infection and controlled human malaria infection (Russell et al., 2011; Shakri et al., 2012; Payne et al., 2017). The adaptation of P. knowlesi to long-term culture (Butcher, 1979) provides a unique platform to explore the basic biology of P. vivax. Further, P. knowlesi can be cultured in human RBC with human serum without the need of rhesus monkey RBC and serum (Moon et al., 2013). The transfection efficiency achieved with this human-adapted P. cynomolgi line is 30%–40% which is 100,000 fold more than that of P. falciparum and surpasses that obtained with P. berghei. Because of the high transfection efficiency, the transgenic parasites can be observed in the first generation itself after transfection. However, the major drawback associated with this line is absence of gametocyte production (Moon et al., 2013).Moreover, Mohring et al. performed clustered regularly interspaced short palindromic repeats (CRISPR-Cas9) mediated genome editing in P. knowlesi. The key parameters involved in CRISPR-Cas9 mediated genome editing were examined and it emerged as a highly robust process with 100% successful transfection (Mohring et al., 2019). This will enable genome-wide systematic knockout or gene tagging in a Plasmodium parasite that can infect human.
Passive merozoite “invasion” in reticulocytes
Despite standardising different aspects, maintaining a continuous P. vivax in vitro culture remains elusive. The lack of continuous in vitro culture is predominantly attributed to the inability of P. vivax merozoites to invade new reticulocytes (Russell et al., 2011; Borlon et al., 2012; Tantular et al., 2012). To overcome the hurdles of successful active merozoite invasion in to reticulocytes, merozoites could be passively introduced into the reticulocytes through electroporation (as in routine transfection procedures) or intracytoplasmic merozoite injection (akin to the procedure of intracytoplasmic sperm injection) and its after-effects be investigated. The size of merozoites is 1–1.5 μm and the diameter of red blood cells is in the range of 6–8 μm which makes it feasible, at least theoretically. It would be interesting to think of a direct delivery of free merozoites in to reticulocytes which can have significant impact on the success of P. vivax in vitro culture. However, the success of such intracytoplasmic delivery of free merozoites in red blood cells would entirely depend on the survival of injected merozoites lacking a parasitophorous vacuolar membrane (PVM). Isolation of viable merozoites has been well established in Plasmodium knowlesi (Dennis et al., 1975; Bannister and Mitchell, 1989; Lyth et al., 2018) and Plasmodium falciparum (Boyle et al., 2010) but it remains unattempted in P. vivax. The purified merozoites remain intact and retained their invasive capacity. The established protocol of merozoite isolation can be utilised for P. vivax merozoites isolation and reticulocyte invasion assays or for direct delivery in reticulocytes.
However, the direct delivery of merozoites in the reticulocyte cytoplasm may not form the PVM and subsequently the parasite may not survive. Although PVM is known to aid the transport of proteins from parasite cytoplasm to the host cell cytoplasm, nutrients uptake and waste excretion (Goldberg and Zimmerberg, 2020), PVM is also shown as non-essential for every intracellular apicomplexans; as some of the parasites belonging to the genus Theileria and Babesia can survive without PVM in the host cytoplasm (Lingelbach and Joiner, 1998; Shaw, 2003; Repnik et al., 2015). Further, the significance of PVM in Pasmodium parasites remains elusive (Goldberg and Zimmerberg, 2020). Merozoites intracytoplasmic injection in reticulocytes might open a door to understand the biology of Plasmodium PVM as well.
Conclusion
In the last 10 decades, several attempts have been made to establish P. vivax in vitro culture with minor achievements. However, significant advancements have been made towards primate-adapted P. vivax in vitro culture. Continuous long-term maintenance of P. vivax in vitro growth with high(er) parasitemia using human isolates is still unachieved and requires due attention. The most enigmatic factor associated with P. vivax in vitro culture is the lack of reproducibility with the published protocol. Golenda et al. (1997) developed the most successful protocol to culture these parasites for 6–8 generation using hemochromatosis patients’ blood. Shaw-Saliba and colleagues reattempted to optimise Golendas’ protocol using P. vivax primate-adapted strain Sal-1 obtained from Aotus lemurinus lemurinus. However, the results of Golenda study was not replicated and the reason could be difference in young reticulocyte contents, iron levels or may be because of differences in strains of P. vivax used. Each human P. vivax isolate has its unique growth kinetics in in vitro system which it could be due to unique genetic makeup of individual isolates and this needs to be explored to improve the current efforts to sustain in vitro P. vivax cultures. Further investigations are required in order to address the variables associated with Golendas’ and other researcher’s study. Moreover, reticulocytes from haemochromatosis patients do support P. vivax growth in vitro may be because of abundance of iron in them, however, reticulocytes’ osmotic stability plays an important role in P. vivax infection that might impact long term P. vivax in vitro culture. The efforts should also include the possibilities of making an immortalised reticulocyte line from erythroid progenitor cells and some blue sky research using passive delivery of P. vivax merozoites into reticulocytes/RBCs.
With regards to transfection success stories, transient transfection has been achieved with P. vivax however, further optimisation is required using human isolates. Genetic manipulation in P. vivax has not been successfully attempted yet. Since lack of continuous culture system is a major limitation in advancement of transfection technologies in P. vivax, the use of alternate Plasmodium species (P. cynomolgi and P. knowlesi) that mimic some of the biology of P. vivax may be encouraged as facultative approaches, till an ideal P. vivax long term culture system is established.
Author contributions
SK drafted and wrote the manuscript. AS conceptualised, designed, edited and reviewed the manuscript. All authors contributed to the article and approved the submitted version.
Funding
The work was funded by Indian Council of Medical Research (ICMR), New Delhi in the form of Postdoctoral fellowship to Sanju Kumari (ICMR-PDF).
Conflict of interest
The authors declare that the research was conducted in the absence of any commercial or financial relationships that could be construed as a potential conflict of interest.
Publisher’s note
All claims expressed in this article are solely those of the authors and do not necessarily represent those of their affiliated organizations, or those of the publisher, the editors and the reviewers. Any product that may be evaluated in this article, or claim that may be made by its manufacturer, is not guaranteed or endorsed by the publisher.
Abbreviations
CD71, Cluster of differentiation 71; CRISPR/Cas9, Clustered regularly interspaced short palindromic repeats/Crispr associated protein9; DMEM, Dulbecco’s medium eagle medium; DMSO, Dimethylsulfoxide; FACS, Flourescence activated cell sorting; G6PD, Glucose 6-phosphate dehydrogenase; HC, Heilmeyer’s class; HEPES, 4-(2-hydroxylethyl)-1-piperazineethanesulfonic acid; PV, Parasitophorous vacuole; Pv-IRBCs, Plasmodium vivax-infected red blood cells; PVM, Parasitophorous vacuolar membrane; Pvdhfr, Plasmodium vivax dihydrofolate reductase; RBC, Red blood cell; ZFN, Zinc finger nuclease.
References
Adams, J. H., Hudson, D. E., Torii, M., Ward, G. E., Wellems, T. E., Aikawa, M., et al. (1990). The Duffy receptor family of Plasmodium knowlesi is located within the micronemes of invasive malaria merozoites. Cells 63, 141–153. doi: 10.1016/0092-8674(90)90295-P
Aikawa, M., Miller, L. H., and Rabbege, J. (1975). Caveola--vesicle complexes in the plasmalemma of erythrocytes infected by Plasmodium vivax and P cynomolgi. Unique structures related to Schuffner's dots. Am. J. Pathol. 79, 285–300.
Akinyi, S., Hanssen, E., Meyer, E. V., Jiang, J., Korir, C. C., Singh, B., et al. (2012). A 95 kDa protein of Plasmodium vivax and P. cynomolgi visualized by three-dimensional tomography in the caveola-vesicle complexes (Schuffner's dots) of infected erythrocytes is a member of the PHIST family. Mol. Microbiol. 84, 816–831. doi: 10.1111/j.1365-2958.2012.08060.x
Alexandre, M. A., Ferreira, C. O., Siqueira, A. M., Magalhaes, B. L., Mourao, M. P., Lacerda, M. V., et al. (2010). Severe Plasmodium vivax malaria, Brazilian Amazon. Emerg. Infect. Dis. 16, 1611–1614. doi: 10.3201/eid1610.100685
Andrews, N. C., Fleming, M. D., and Levy, J. E. (1999). Molecular insights into mechanisms of iron transport. Curr. Opin. Hematol. 6, 61–64. doi: 10.1097/00062752-199903000-00001
Anstey, N. M., Handojo, T., Pain, M. C., Kenangalem, E., Tjitra, E., Price, R. N., et al. (2007). Lung injury in vivax malaria: pathophysiological evidence for pulmonary vascular sequestration and posttreatment alveolar-capillary inflammation. J. Infect. Dis. 195, 589–596. doi: 10.1086/510756
Anstey, N. M., Russell, B., Yeo, T. W., and Price, R. N. (2009). The pathophysiology of vivax malaria. Trends Parasitol. 25, 220–227. doi: 10.1016/j.pt.2009.02.003
Azevedo, M. F., and del Portillo, H. A. (2007). Promoter regions of Plasmodium vivax are poorly or not recognized by Plasmodium falciparum. Malar. J. 6:20. doi: 10.1186/1475-2875-6-20
Baird, J. K. (2007). Neglect of Plasmodium vivax malaria. Trends Parasitol. 23, 533–539. doi: 10.1016/j.pt.2007.08.011
Bannister, L. H., and Mitchell, G. H. (1989). The fine structure of secretion by Plasmodium knowlesi merozoites during red cell invasion. J. Protozool. 36, 362–367. doi: 10.1111/j.1550-7408.1989.tb05527.x
Barnwell, J. W., Nichols, M. E., and Rubinstein, P. (1989). In vitro evaluation of the role of the Duffy blood group in erythrocyte invasion by Plasmodium vivax. J. Exp. Med. 169, 1795–1802. doi: 10.1084/jem.169.5.1795
Baro, B., Deroost, K., Raiol, T., Brito, M., Almeida, A. C. G., de Menezes-Neto, A., et al. (2017). Plasmodium vivax gametocytes in the bone marrow of an acute malaria patient and changes in the erythroid miRNA profile. PLoS Negl. Trop. Dis. 11:e0005365. doi: 10.1371/journal.pntd.0005365
Bass, C. C., and Johns, F. M. (1912). The cultivation of malarial plasmodia (Plasmodium vivax and Plasmodium falciparum) in vitro. J. Exp. Med. 16, 567–579. doi: 10.1084/jem.16.4.567
Bermudez, M., Moreno-Perez, D. A., Arevalo-Pinzon, G., Curtidor, H., and Patarroyo, M. A. (2018). Plasmodium vivax in vitro continuous culture: the spoke in the wheel. Malar. J. 17:301. doi: 10.1186/s12936-018-2456-5
Borlon, C., Russell, B., Sriprawat, K., Suwanarusk, R., Erhart, A., Renia, L., et al. (2012). Cryopreserved Plasmodium vivax and cord blood reticulocytes can be used for invasion and short term culture. Int. J. Parasitol. 42, 155–160. doi: 10.1016/j.ijpara.2011.10.011
Bourgard, C., Albrecht, L., Kayano, A., Sunnerhagen, P., and Costa, F. T. M. (2018). Plasmodium vivax biology: insights provided by genomics, transcriptomics and proteomics. Front. Cell. Infect. Microbiol. 8:34. doi: 10.3389/fcimb.2018.00034
Boyd, M. F., and Kitchen, S. (1937). Simultaneous inoculation with Plasmodium vivax and Plasmodium falciparum1. Am. J. Trop. Med. Hygiene. 1, 855–861.
Boyle, M. J., Wilson, D. W., Richards, J. S., Riglar, D. T., Tetteh, K. K., Conway, D. J., et al. (2010). Isolation of viable plasmodium falciparum merozoites to define erythrocyte invasion events and advance vaccine and drug development. Proc. Natl. Acad. Sci. U. S. A. 107, 14378–14383. doi: 10.1073/pnas.1009198107
Butcher, G. A. (1979). Factors affecting the in vitro culture of plasmodium falciparum and plasmodium knowlesi. Bull. World Health Organ. 57, 17–26.
Chua, A. C., Ong, J. J., Malleret, B., Suwanarusk, R., Kosaisavee, V., Zeeman, A. M., et al. (2019). Robust continuous in vitro culture of the Plasmodium cynomolgi erythrocytic stages. Nat. Commun. 10, 1–3. doi: 10.1038/s41467-019-11332-4
Clark, M. A., Kanjee, U., Rangel, G. W., Chery, L., Mascarenhas, A., Gomes, E., et al. (2021). Plasmodium vivax infection compromises reticulocyte stability. Nat. Commun. 12:1629. doi: 10.1038/s41467-021-21886-x
Cogswell, F. B. (1992). The hypnozoite and relapse in primate malaria. Clin. Microbiol. Rev. 5, 26–35. doi: 10.1128/CMR.5.1.26
Collins, W. E., Jeffery, G. M., and Roberts, J. M. (2003). A retrospective examination of anemia during infection of humans with Plasmodium vivax. Am. J. Trop. Med. Hyg. 68, 410–412. doi: 10.4269/ajtmh.2003.68.410
Colpo, E., de Bem, A. F., Pieniz, S., Schettert, S. D., dos Santos, R. M., Farias, I. L., et al. (2008). A single high dose of ascorbic acid and iron is not correlated with oxidative stress in healthy volunteers. Ann. Nutr. Metab. 53, 79–85. doi: 10.1159/000162257
Commons, R. J., Simpson, J. A., Thriemer, K., Hossain, M. S., Douglas, N. M., Humphreys, G. S., et al. (2019). Risk of Plasmodium vivax parasitaemia after plasmodium falciparum infection: a systematic review and meta-analysis. Lancet Infect. Dis. 19, 91–101. doi: 10.1016/S1473-3099(18)30596-6
Conrad, Jr, M. E., Berman, A., and Crosby, W. H. (1962). Iron kinetics in Laennec’s cirrhosis. Gastroenterology 43, 385–390.
Costa, F. T., Lopes, S. C., Ferrer, M., Leite, J. A., Martin-Jaular, L., Bernabeu, M., et al. (2011). On cytoadhesion of Plasmodium vivax: raison d'etre? Mem. Inst. Oswaldo Cruz 106, 79–84. doi: 10.1590/S0074-02762011000900010
Crabb, B. S., Rug, M., Gilberger, T. W., Thompson, J. K., Triglia, T., Maier, A. G., et al. (2004). Transfection of the human malaria parasite Plasmodium falciparum. Methods Mol. Biol. 270, 263–276. doi: 10.1385/1-59259-793-9:263
Crabb, B. S., Triglia, T., Waterkeyn, J. G., and Cowman, A. F. (1997). Stable transgene expression in Plasmodium falciparum. Mol. Biochem. Parasitol. 90, 131–144. doi: 10.1016/S0166-6851(97)00143-6
Daniels, D. E., Ferguson, D. C., Griffiths, R. E., Trakarnsanga, K., Cogan, N., MacInnes, K. A., et al. (2021). Reproducible immortalization of erythroblasts from multiple stem cell sources provides approach for sustainable RBC therapeutics. Mol. Ther.-Methods Clin. Dev. 22, 26–39. doi: 10.1016/j.omtm.2021.06.002
de Koning-Ward, T. F., Fidock, D. A., Thathy, V., Menard, R., van Spaendonk, R. M., Waters, A. P., et al. (2000). The selectable marker human dihydrofolate reductase enables sequential genetic manipulation of the plasmodium berghei genome. Mol. Biochem. Parasitol. 106, 199–212. doi: 10.1016/S0166-6851(99)00189-9
del Portillo, H. A., Lanzer, M., Rodriguez-Malaga, S., Zavala, F., and Fernandez-Becerra, C. (2004). Variant genes and the spleen in Plasmodium vivax malaria. Int. J. Parasitol. 34, 1547–1554. doi: 10.1016/j.ijpara.2004.10.012
Dennis, E. D., Mitchell, G. H., Butcher, G. A., and Cohen, S. (1975). In vitro isolation of Plasmodium knowlesi merozoites using polycarbonate sieves. Parasitology 71, 475–481. doi: 10.1017/S0031182000047235
Douglas, N. M., Lampah, D. A., Kenangalem, E., Simpson, J. A., Poespoprodjo, J. R., Sugiarto, P., et al. (2013). Major burden of severe anemia from non-falciparum malaria species in southern Papua: a hospital-based surveillance study. PLoS Med. 10:e1001575:e1001575; discussion e1001575. doi: 10.1371/journal.pmed.1001575
Douglas, N. M., Nosten, F., Ashley, E. A., Phaiphun, L., van Vugt, M., Singhasivanon, P., et al. (2011). Plasmodium vivax recurrence following falciparum and mixed species malaria: risk factors and effect of antimalarial kinetics. Clin. Infect. Dis. 52, 612–620. doi: 10.1093/cid/ciq249
Douglas, N. M., Pontororing, G. J., Lampah, D. A., Yeo, T. W., Kenangalem, E., Poespoprodjo, J. R., et al. (2014). Mortality attributable to Plasmodium vivax malaria: a clinical audit from Papua, Indonesia. BMC Medicine. 12, 1–3. doi: 10.1186/s12916-014-0217-z
Feeney, G. P., Carter, K., Masters, G. S., Jackson, H. A., Cavil, I., and Worwood, M. (2005). Changes in erythropoiesis in hereditary hemochromatosis are not mediated by HFE expression in nucleated red cells. Haematologica 90, 180–187.
Fernandez-Becerra, C., Lelievre, J., Ferrer, M., Anton, N., Thomson, R., Peligero, C., et al. (2013). Red blood cells derived from peripheral blood and bone marrow CD34(+) human haematopoietic stem cells are permissive to plasmodium parasites infection. Mem. Inst. Oswaldo Cruz 108, 801–803. doi: 10.1590/0074-0276108062013019
Field, J. W., Sandosham, A. A., and Fong, Y. L. (1963). The microscopial diagnosis of human malaria, Kuala Lampur: The Government Press, 20–117.
Field, J. W., and Shute, P. G. (1956). The microscopic diagnosis of human malaria. II. A Morphological Study of the Erythroeytic Parasites.(24).
Gildersleeve, R. P., Thaxton, J. P., Galvin, M. J., and McRee, D. I. (1985). Hematological response of immunized and serially bled Japanese quail. Poultry Science 64, 1806–1808.
Goldberg, D. E., and Zimmerberg, J. (2020). Hardly vacuous: the Parasitophorous vacuolar membrane of malaria parasites. Trends Parasitol. 36, 138–146. doi: 10.1016/j.pt.2019.11.006
Golenda, C. F., Li, J., and Rosenberg, R. (1997). Continuous in vitro propagation of the malaria parasite Plasmodium vivax. Proc. Natl. Acad. Sci. U. S. A. 94, 6786–6791. doi: 10.1073/pnas.94.13.6786
Grüring, C., Moon, R. W., Lim, C., Holder, A. A., Blackman, M. J., and Duraisingh, M. T. (2014). Human red blood cell-adapted Plasmodium knowlesi parasites: a new model system for malaria research. Cell. Microbiol. 16, 612–620. doi: 10.1111/cmi.12275
Gruszczyk, J., Kanjee, U., Chan, L. J., Menant, S., Malleret, B., Lim, N. T. Y., et al. (2018). Transferrin receptor 1 is a reticulocyte-specific receptor for Plasmodium vivax. Science 359, 48–55. doi: 10.1126/science.aan1078
Gunalan, K., Rowley, E. H., and Miller, L. H. (2020). A way forward for culturing Plasmodium vivax. Trends Parasitol. 36, 512–519. doi: 10.1016/j.pt.2020.04.002
Handayani, S., Chiu, D. T., Tjitra, E., Kuo, J. S., Lampah, D., Kenangalem, E., et al. (2009). High deformability of Plasmodium vivax-infected red blood cells under microfluidic conditions. J. Infect. Dis. 199, 445–450. doi: 10.1086/596048
Heilmeyer, L., and Westhäuser, R. (1932). Reifungsstadien an überlebenden reticulozyten in vitro und ihre bedeutung für die schaetzung der täglichen haemoglobin-produktion in vivo. Ztschr. Klin. Med. 121, 361–379.
Hirose, S. I., Takayama, N., Nakamura, S., Nagasawa, K., Ochi, K., Hirata, S., et al. (2013). Immortalization of erythroblasts by c-MYC and BCL-XL enables large-scale erythrocyte production from human pluripotent stem cells. Stem Cell Reports. 1, 499–508. doi: 10.1016/j.stemcr.2013.10.010
Hossain, M. S., Commons, R. J., Douglas, N. M., Thriemer, K., Alemayehu, B. H., Amaratunga, C., et al. (2020). The risk of Plasmodium vivax parasitemia after P. falciparum malaria: an individual patient data meta-analysis from the WorldWide antimalarial resistance network. PLoS Med. 17:e1003393. doi: 10.1371/journal.pmed.1003393
Hu, Z., Van Rooijen, N., and Yang, Y. G. (2011). Macrophages prevent human red blood cell reconstitution in immunodeficient mice. Blood 118, 5938–5946. doi: 10.1182/blood-2010-11-321414
Huang, X., Shah, S., Wang, J., Ye, Z., Dowey, S. N., Tsang, K. M., et al. (2014). Extensive ex vivo expansion of functional human erythroid precursors established from umbilical cord blood cells by defined factors. Mol. Ther. 22, 451–463. doi: 10.1038/mt.2013.201
Jandl, J. H., Inman, J. K., Simmons, R. L., and Allen, D. W. (1959). Transfer of iron from serum iron-binding protein to human reticulocytes. J. Clin. Invest. 38, 161–185. doi: 10.1172/JCI103786
Kanjee, U., Grüring, C., Babar, P., Meyers, A., Dash, R., Pereira, L., et al. (2021). Plasmodium vivax strains use alternative pathways for invasion. J. Infect. Dis. 223, 1817–1821. doi: 10.1093/infdis/jiaa592
Kaufman, R. (2017). “Red blood cell life span, senescence, and destruction” in Anemia: pathophysiology, diagnosis, and management. eds. E. Benz Jr., N. Berliner, and F. Schiffman (Cambridge: Cambridge University Press), 19–22.
Kenangalem, E., Karyana, M., Burdarm, L., Yeung, S., Simpson, J. A., Tjitra, E., et al. (2016). Plasmodium vivax infection: a major determinant of severe anaemia in infancy. Malar. J. 15:321. doi: 10.1186/s12936-016-1373-8
King, C. L., Adams, J. H., Xianli, J., Grimberg, B. T., McHenry, A. M., Greenberg, L. J., et al. (2011). Fya/Fyb antigen polymorphism in human erythrocyte Duffy antigen affects susceptibility to Plasmodium vivax malaria. Proc. Natl. Acad. Sci. U. S. A. 108, 20113–20118. doi: 10.1073/pnas.1109621108
Kitchen, S. (1938). The infection of Retieulocytes by Plasmodium vivax. Am. J. Trop. Med. s1-18, 347–359. doi: 10.4269/ajtmh.1938.s1-18.347
Kochar, D. K., Das, A., Kochar, S. K., Saxena, V., Sirohi, P., Garg, S., et al. (2009). Severe Plasmodium vivax malaria: a report on serial cases from Bikaner in northwestern India. Am J Trop Med Hyg. 80, 194–198. doi: 10.4269/ajtmh.2009.80.194
Kochar, D. K., Saxena, V., Singh, N., Kochar, S. K., Kumar, S. V., and Das, A. (2005). Plasmodium vivax malaria. Emerg. Infect. Dis. 11, 132–134. doi: 10.3201/eid1101.040519
Kocken, C. H., Ozwara, H., van der Wel, A., Beetsma, A. L., Mwenda, J. M., and Thomas, A. W. (2002). Plasmodium knowlesi provides a rapid in vitro and in vivo transfection system that enables double-crossover gene knockout studies. Infect. Immun. 70, 655–660. doi: 10.1128/IAI.70.2.655-660.2002
Kocken, C. H., van der Wel, A., and Thomas, A. W. (1999). Plasmodium cynomolgi: transfection of blood-stage parasites using heterologous DNA constructs. Exp. Parasitol. 93, 58–60. doi: 10.1006/expr.1999.4430
Kono, M., Kondo, T., Takagi, Y., Wada, A., and Fujimoto, K. (2009). Morphological definition of CD71 positive reticulocytes by various staining techniques and electron microscopy compared to reticulocytes detected by an automated hematology analyzer. Clin. Chim. Acta 404, 105–110. doi: 10.1016/j.cca.2009.03.017
Krotoski, W. A. (1985). Discovery of the hypnozoite and a new theory of malarial relapse. Trans. R. Soc. Trop. Med. Hyg. 79, 1–11. doi: 10.1016/0035-9203(85)90221-4
Kurita, R., Suda, N., Sudo, K., Miharada, K., Hiroyama, T., Miyoshi, H., et al. (2013). Establishment of immortalized human erythroid progenitor cell lines able to produce enucleated red blood cells. PLoS One 8:e59890. doi: 10.1371/journal.pone.0059890
Lanners, H. N. (1992). Prolonged in vitro cultivation of Plasmodium vivax using Trager's continuous-flow method. Parasitol. Res. 78, 699–701. doi: 10.1007/BF00931524
Larrouy, G., Magnaval, J. F., and Moro, F. (1981). Obtaining intraerythrocytic forms of Plasmodium vivax by in vitro culture. C. R. Seances Acad. Sci. III 292, 929–930.
Lim, C., Pereira, L., Saliba, K. S., Mascarenhas, A., Maki, J. N., Chery, L., et al. (2016). Reticulocyte preference and stage development of Plasmodium vivax isolates. J. Infect. Dis. 214, 1081–1084. doi: 10.1093/infdis/jiw303
Lingelbach, K., and Joiner, K. A. (1998). The Parasitophorous vacuole membrane surrounding plasmodium and toxoplasma: an unusual compartment in infected cells. J. Cell Sci. 111, 1467–1475. doi: 10.1242/jcs.111.11.1467
Lopes, S. C., Albrecht, L., Carvalho, B. O., Siqueira, A. M., Thomson-Luque, R., Nogueira, P. A., et al. (2014). Paucity of Plasmodium vivax mature schizonts in peripheral blood is associated with their increased cytoadhesive potential. J. Infect. Dis. 209, 1403–1407. doi: 10.1093/infdis/jiu018
Luiza-Batista, C., Thiberge, S., Serra-Hassoun, M., Nardella, F., Claës, A., Nicolete, V. C., et al. (2022). Humanized mice for investigating sustained Plasmodium vivax blood-stage infections and transmission. Nat. Commun. 13, 1–9. doi: 10.1038/s41467-022-31864-6
Luzzatto, L. (2006). Glucose 6-phosphate dehydrogenase deficiency: from genotype to phenotype. Haematologica 91, 1303–1306. doi: 10.3324/%25x
Lyth, O., Vizcay-Barrena, G., Wright, K. E., Haase, S., Mohring, F., Najer, A., et al. (2018). Cellular dissection of malaria parasite invasion of human erythrocytes using viable Plasmodium knowlesi merozoites. Sci. Rep. 8:1. doi: 10.1038/s41598-018-28457-z
Malleret, B., Li, A., Zhang, R., Tan, K. S., Suwanarusk, R., Claser, C., et al. (2015). Plasmodium vivax: restricted tropism and rapid remodeling of CD71-positive reticulocytes. Blood 125, 1314–1324. doi: 10.1182/blood-2014-08-596015
Malleret, B., Xu, F., Mohandas, N., Suwanarusk, R., Chu, C., Leite, J. A., et al. (2013). Significant biochemical, biophysical and metabolic diversity in circulating human cord blood reticulocytes. PLoS One 8:e76062. doi: 10.1371/journal.pone.0076062
McKenzie, F. E., Jeffery, G. M., and Collins, W. E. (2002). Plasmodium vivax blood-stage dynamics. J. Parasitol. 88, 521–535. doi: 10.1645/0022-3395(2002)088[0521:PVBSD]2.0.CO;2
Mehlotra, R. K., Blankenship, D., Howes, R. E., Rakotomanga, T. A., Ramiranirina, B., Ramboarina, S., et al. (2017). Long-term in vitro culture of Plasmodium vivax isolates from Madagascar maintained in Saimiri boliviensis blood. Malar. J. 16:442. doi: 10.1186/s12936-017-2090-7
Miao, J., and Cui, L. (2011). Rapid isolation of single malaria parasite-infected red blood cells by cell sorting. Nat. Protoc. 6, 140–146. doi: 10.1038/nprot.2010.185
Mohapatra, M. K., Padhiary, K. N., Mishra, D. P., and Sethy, G. (2002). Atypical manifestations of Plasmodium vivax malaria. Indian J. Malariol. 39, 18–25.
Mohring, F., Hart, M. N., Rawlinson, T. A., Henrici, R., Charleston, J. A., Diez Benavente, E., et al. (2019). Rapid and iterative genome editing in the malaria parasite Plasmodium knowlesi provides new tools for P. vivax research. eLife 8:8. doi: 10.7554/eLife.45829
Mons, B. (1990). Preferential invasion of malarial merozoites into young red blood cells. Blood Cells 16, 299–312.
Moon, R. W., Hall, J., Rangkuti, F., Ho, Y. S., Almond, N., Mitchell, G. H., et al. (2013). Adaptation of the genetically tractable malaria pathogen plasmodium knowlesi to continuous culture in human erythrocytes. Proc. Natl. Acad. Sci. U. S. A. 110, 531–536. doi: 10.1073/pnas.1216457110
Moraes Barros, R. R., Straimer, J., Sa, J. M., Salzman, R. E., Melendez-Muniz, V. A., Mu, J., et al. (2015). Editing the Plasmodium vivax genome, using zinc-finger nucleases. J. Infect. Dis. 211, 125–129. doi: 10.1093/infdis/jiu423
Mueller, I., Galinski, M. R., Baird, J. K., Carlton, J. M., Kochar, D. K., Alonso, P. L., et al. (2009). Key gaps in the knowledge of Plasmodium vivax, a neglected human malaria parasite. Lancet Infect. Dis. 9, 555–566. doi: 10.1016/S1473-3099(09)70177-X
Noulin, F., Borlon, C., van den Eede, P., Boel, L., Verfaillie, C. M., D'Alessandro, U., et al. (2012). Cryopreserved reticulocytes derived from hematopoietic stem cells can be invaded by cryopreserved Plasmodium vivax isolates. PLoS One 7:e40798. doi: 10.1371/journal.pone.0040798
Noulin, F., Manesia, J. K., Rosanas-Urgell, A., Erhart, A., Borlon, C., Van Den Abbeele, J., et al. (2014). Hematopoietic stem/progenitor cell sources to generate reticulocytes for Plasmodium vivax culture. PLoS One 9:e112496. doi: 10.1371/journal.pone.0112496
Panichakul, T., Sattabongkot, J., Chotivanich, K., Sirichaisinthop, J., Cui, L., and Udomsangpetch, R. (2007). Production of erythropoietic cells in vitro for continuous culture of Plasmodium vivax. Int. J. Parasitol. 37, 1551–1557. doi: 10.1016/j.ijpara.2007.05.009
Pasini, E. M., Böhme, U., Rutledge, G. G., Voorberg-Van der Wel, A., Sanders, M., Berriman, M., et al. (2017). An improved Plasmodium cynomolgi genome assembly reveals an unexpected methy ltransferase gene expansion. Wellcome Open Res. 2:2. doi: 10.12688/wellcomeopenres.11864.1
Patriani, D., Arguni, E., Kenangalem, E., Dini, S., Sugiarto, P., Hasanuddin, A., et al. (2019). Early and late mortality after malaria in young children in Papua, Indonesia. BMC Infect. Dis. 19, 1–3. doi: 10.1186/s12879-019-4497-y
Payne, R. O., Griffin, P. M., McCarthy, J. S., and Draper, S. J. (2017). Plasmodium vivax controlled human malaria infection – progress and prospects. Trends Parasitol. 33, 141–150. doi: 10.1016/j.pt.2016.11.001
Pfahler, J. M., Galinski, M. R., Barnwell, J. W., and Lanzer, M. (2006). Transient transfection of Plasmodium vivax blood stage parasites. Mol. Biochem. Parasitol. 149, 99–101. doi: 10.1016/j.molbiopara.2006.03.018
Price, L., Planche, T., Rayner, C., and Krishna, S. (2007). Acute respiratory distress syndrome in Plasmodium vivax malaria: case report and review of the literature. Trans. R. Soc. Trop. Med. Hyg. 101, 655–659. doi: 10.1016/j.trstmh.2007.02.014
Punnath, K., Dayanand, K. K., Chandrashekar, V. N., Achur, R. N., Kakkilaya, S. B., Ghosh, S. K., et al. (2020). Clinical features and haematological parameters among malaria patients in Mangaluru city area in the southwestern coastal region of India. Parasitol. Res. 119, 1043–1056. doi: 10.1007/s00436-019-06540-2
Puntarulo, S. (2005). Iron, oxidative stress and human health. Mol. Asp. Med. 26, 299–312. doi: 10.1016/j.mam.2005.07.001
Rangel, G. W., Clark, M. A., Kanjee, U., Goldberg, J. M., MacInnis, B., José Menezes, M., et al. (2020). Plasmodium vivax transcriptional profiling of low input cryopreserved isolates through the intraerythrocytic development cycle. PLoS Negl. Trop. Dis. 14:e0008104. doi: 10.1371/journal.pntd.0008104
Rangel, G. W., Clark, M. A., Kanjee, U., Lim, C., Shaw-Saliba, K., Menezes, M. J., et al. (2018). Enhanced ex vivo Plasmodium vivax intraerythrocytic enrichment and maturation for rapid and sensitive parasite growth assays. Antimicrob. Agents Chemother. 62, e02519–e02517. doi: 10.1128/AAC.02519-17
Repnik, U., Gangopadhyay, P., Bietz, S., Przyborski, J. M., Griffiths, G., and Lingelbach, K. (2015). The apicomplexan parasite Babesia divergens internalizes band 3, glycophorin a and spectrin during invasion of human red blood cells. Cell. Microbiol. 17, 1052–1068. doi: 10.1111/cmi.12422
Riley, R. S., Ben-Ezra, J. M., Goel, R., and Tidwell, A. (2001). Reticulocytes and reticulocyte enumeration. J. Clin. Lab. Anal. 15, 267–294. doi: 10.1002/jcla.1039
Roobsoong, W., Tharinjaroen, C. S., Rachaphaew, N., Chobson, P., Schofield, L., Cui, L., et al. (2015). Improvement of culture conditions for long-term in vitro culture of Plasmodium vivax. Malar. J. 14:297. doi: 10.1186/s12936-015-0815-z
Rudolf, G. D. M., and Ramsay, J. (1927). Enumeration of parasites in therapeutic malaria. J. Tropical Med. Hygiene. 30, 1–8.
Russell, B., Suwanarusk, R., Borlon, C., Costa, F. T., Chu, C. S., Rijken, M. J., et al. (2011). A reliable ex vivo invasion assay of human reticulocytes by Plasmodium vivax. Blood 118, e74–e81. doi: 10.1182/blood-2011-04-348748
Shakri, A. R., Rizvi, M. M., and Chitnis, C. E. (2012). Development of quantitative receptor-ligand binding assay for use as a tool to estimate immune responses against Plasmodium vivax duffy binding protein region II. J. Immunoassay Immunochem. 33, 403–413. doi: 10.1080/15321819.2012.659781
Shanks, G. D., and White, N. J. (2013). The activation of vivax malaria hypnozoites by infectious diseases. Lancet Infect. Dis. 13, 900–906. doi: 10.1016/S1473-3099(13)70095-1
Shaw, M. K. (2003). Cell invasion by Theileria sporozoites. Trends Parasitol. 19, 2–6. doi: 10.1016/S1471-4922(02)00015-6
Shaw-Saliba, K., Thomson-Luque, R., Obaldia, N. 3rd, Nunez, M., Dutary, S., Lim, C., et al. (2016). Insights into an optimization of Plasmodium vivax Sal-1 in vitro culture: the Aotus primate model. PLoS Negl. Trop. Dis. 10:e0004870. doi: 10.1371/journal.pntd.0004870
Siddiqui, W. A. (1979). In vitro cultivation of Plasmodium vivax and Plasmodium malariae. Cambridge: Adacemic Press. 279–285.
Singh, G., Urhekar, A., and Singh, R. (2015). In vitro cultivation of Plasmodium vivax using McCoy’s medium. Asian J. Med. Pharm. Res. 5, 18–21.
Suwanarusk, R., Cooke, B. M., Dondorp, A. M., Silamut, K., Sattabongkot, J., White, N. J., et al. (2004). The deformability of red blood cells parasitized by Plasmodium falciparum and P. vivax. J. Infect. Dis. 189, 190–194. doi: 10.1086/380468
Tachibana, S., Sullivan, S. A., Kawai, S., Nakamura, S., Kim, H. R., Goto, N., et al. (2012). Plasmodium cynomolgi genome sequences provide insight into Plasmodium vivax and the monkey malaria clade. Nat. Genet. 44, 1051–1055. doi: 10.1038/ng.2375
Tantular, I. S., Pusarawati, S., Khin, L., Kanbe, T., Kimura, M., Kido, Y., et al. (2012). Preservation of wild isolates of human malaria parasites in wet ice and adaptation efficacy to in vitro culture. Trop Med Health. 40, 37–45. doi: 10.2149/tmh.2012-07o
Thawnashom, K., Kaneko, M., Xangsayarath, P., Chaiyawong, N., Yahata, K., Asada, M., et al. (2019). Validation of Plasmodium vivax centromere and promoter activities using Plasmodium yoelii. PLoS One 14:e0226884. doi: 10.1371/journal.pone.0226884
Thomson-Luque, R., Adams, J. H., Kocken, C. H. M., and Pasini, E. M. (2019). From marginal to essential: the golden thread between nutrient sensing, medium composition and Plasmodium vivax maturation in in vitro culture. Malar. J. 18:344. doi: 10.1186/s12936-019-2949-x
Thomson-Luque, R., and Bautista, J. M. (2021). Home sweet home: Plasmodium vivax-infected reticulocytes-the younger the better? Front. Cell. Infect. Microbiol. 11:675156. doi: 10.3389/fcimb.2021.675156
Thomson-Luque, R., Shaw Saliba, K., Kocken, C. H. M., and Pasini, E. M. (2017). A continuous, long-term Plasmodium vivax in vitro blood-stage culture: what are we missing? Trends Parasitol. 33, 921–924. doi: 10.1016/j.pt.2017.07.001
Toda, H., Diaz-Varela, M., Segui-Barber, J., Roobsoong, W., Baro, B., Garcia-Silva, S., et al. (2020). Plasma-derived extracellular vesicles from Plasmodium vivax patients signal spleen fibroblasts via NF-kB facilitating parasite cytoadherence. Nat. Commun. 11:2761. doi: 10.1038/s41467-020-16337-y
Trager, W., and Jensen, J. B. (1976). Human malaria parasites in continuous culture. Science 193, 673–675. doi: 10.1126/science.781840
Trakarnsanga, K., Griffiths, R. E., Wilson, M. C., Blair, A., Satchwell, T. J., Meinders, M., et al. (2017). An immortalized adult human erythroid line facilitates sustainable and scalable generation of functional red cells. Nat. Commun. 8, 1–7. doi: 10.1038/ncomms14750
Udomsangpetch, R., Somsri, S., Panichakul, T., Chotivanich, K., Sirichaisinthop, J., Yang, Z., et al. (2007). Short-term in vitro culture of field isolates of Plasmodium vivax using umbilical cord blood. Parasitol. Int. 56, 65–69. doi: 10.1016/j.parint.2006.12.005
van der Wel, A. M., Tomas, A. M., Kocken, C. H., Malhotra, P., Janse, C. J., Waters, A. P., et al. (1997). Transfection of the primate malaria parasite Plasmodium knowlesi using entirely heterologous constructs. J. Exp. Med. 185, 1499–1504. doi: 10.1084/jem.185.8.1499
Wilson, M. C., Trakarnsanga, K., Heesom, K. J., Cogan, N., Green, C., Toye, A. M., et al. (2016). Comparison of the proteome of adult and cord Erythroid cells, and changes in the proteome following reticulocyte maturation. Mol. Cell. Proteomics 15, 1938–1946. doi: 10.1074/mcp.M115.057315
World Health Organization (2021). World malaria report 2021—WHO (World Health Organization). Available at: https://www.who.int/publications/i/item/9789240040496
Keywords: Plasmodium vivax, reticulocytes, in vitro culture, transfection, malaria
Citation: Kumari S and Sinha A (2023) Culture and transfection: Two major bottlenecks in understanding Plasmodium vivax biology. Front. Microbiol. 14:1144453. doi: 10.3389/fmicb.2023.1144453
Edited by:
Suvankar Ghorai, Raiganj University, IndiaReviewed by:
Katy Shaw-Saliba, National Institute of Allergy and Infectious Diseases (NIH), United StatesCopyright © 2023 Kumari and Sinha. This is an open-access article distributed under the terms of the Creative Commons Attribution License (CC BY). The use, distribution or reproduction in other forums is permitted, provided the original author(s) and the copyright owner(s) are credited and that the original publication in this journal is cited, in accordance with accepted academic practice. No use, distribution or reproduction is permitted which does not comply with these terms.
*Correspondence: Abhinav Sinha, abhinavsinha@icmr.gov.in