- 1Integrated Biomarker Research Group, René Rachou Institute, Fiocruz Minas, Oswaldo Cruz Foundation, Belo Horizonte, Minas Gerais, Brazil
- 2Department of Pharmacy, Federal University of the Jequitinhonha and Mucuri Valleys, Diamantina, Minas Gerais, Brazil
Chagas disease, caused by Trypanosoma cruzi, remains a serious public health problem worldwide. The parasite was subdivided into six distinct genetic groups, called “discrete typing units” (DTUs), from TcI to TcVI. Several studies have indicated that the heterogeneity of T. cruzi species directly affects the diversity of clinical manifestations of Chagas disease, control, diagnosis performance, and susceptibility to treatment. Thus, this review aims to describe how T. cruzi genetic diversity influences the biology of the parasite and/or clinical parameters in humans. Regarding the geographic dispersion of T. cruzi, evident differences were observed in the distribution of DTUs in distinct areas. For example, TcII is the main DTU detected in Brazilian patients from the central and southeastern regions, where there are also registers of TcVI as a secondary T. cruzi DTU. An important aspect observed in previous studies is that the genetic variability of T. cruzi can impact parasite infectivity, reproduction, and differentiation in the vectors. It has been proposed that T. cruzi DTU influences the host immune response and affects disease progression. Genetic aspects of the parasite play an important role in determining which host tissues will be infected, thus heavily influencing Chagas disease’s pathogenesis. Several teams have investigated the correlation between T. cruzi DTU and the reactivation of Chagas disease. In agreement with these data, it is reasonable to suppose that the immunological condition of the patient, whether or not associated with the reactivation of the T. cruzi infection and the parasite strain, may have an important role in the pathogenesis of Chagas disease. In this context, understanding the genetics of T. cruzi and its biological and clinical implications will provide new knowledge that may contribute to additional strategies in the diagnosis and clinical outcome follow-up of patients with Chagas disease, in addition to the reactivation of immunocompromised patients infected with T. cruzi.
1 Introduction
Chagas disease, caused by the protozoan Trypanosoma cruzi, was discovered over a century ago and remains a serious public health concern worldwide (1). According to the World Health Organization (WHO), the disease affects approximately 6–7 million people, mainly in Latin America, with 75 million people at risk of infection and more than 10,000 deaths per year (2, 3).
T. cruzi is a flagellate digenetic protozoan belonging to the order Kinetoplastida and family Trypanosomatidae (1). This species is heterogeneous and consists of several subpopulations that circulate in diverse environments, presenting great genetic diversity (4, 5). The analysis of a large panel of clones derived from wild-type isolates confirmed that the genome size can vary up to 48% between T. cruzi strains, and this difference is significant for populations of the same species (6). Hybridization events have contributed to current T. cruzi population structures and distinct genetic subgroups’ evolution of the parasite (4, 7).
These T. cruzi subgroups have been studied with different molecular tools over the years and have received distinct designations, including zymodemes (8–10), schizodemes (11), biodemes (12, 13), clones (14), groups (15), lineages (16), clades (17), and discrete typing units (DTUs) (18). In a Satellite Meeting in 1999, the standardization of T. cruzi strain nomenclature was discussed to adopt a standard classification that taxonomists and the general community of researchers would use. An expert committee reviewed the subdivision of the T. cruzi strains considering biological, biochemical, and molecular parameters to classify the species into two principal groups: T. cruzi I and T. cruzi II. The apparent hybrid strain was named T. cruzi (19).
However, with the advances in molecular biology and knowledge of parasite biology, this classification showed limitations due to the great dispersion of characteristics between members of the same T. cruzi genetic group (20). In the last decades, several molecular approaches have been used to elucidate the population structure of T. cruzi and have revealed a variable number of relevant subgroups in T. cruzi species (21–27). These data suggest that investigations employing the smaller T. cruzi subdivisions present more specific correlations between genetic parasite and biological, clinical, and epidemiological properties of the Chagas disease (Table 1). Consequently, 10 years after the Satellite Meeting, the scientific community has advanced knowledge of the diversity of T. cruzi. Thus, the current classification consensus proposes six genetic groups or DTUs, referred to as TcI to TcVI (38), related to previous classifications according to different markers. A DTU restricted to bats (Tcbat) was also considered (5). Studies based on multiple molecular markers support the definitive classification of Tcbat as a seventh DTU closely related to TcI, mainly found in bats (39, 40) and registered in Colombia, Panama, Chile, Equator, and Brazil (36, 41, 42). TcI and TcII represent ancestral T. cruzi lineages, which, from hybridization events, give rise to TcIII and TcIV, respectively (43, 44). In contrast, Freitas et al. (2006) (26) suggested the existence of three ancestral T. cruzi lineages (TcI, TcII, and TcIII). Nonetheless, genetic analyses showed TcI and TcII traits in TcIV and TcIII isolates, supporting the first model (7, 43, 45). TcV and TcVI are considered genetic hybrid groups formed from TcII and TcIII, which are widely circulated in the domestic cycle in South American countries (46–49). However, more recently, they have been found to infect dogs in the United States (50).
Several studies have shown that the heterogeneity of T. cruzi species directly affects the diversity of clinical manifestations, the control, the laboratorial diagnosis performance, and the susceptibility to treatment in the Chagas disease (Table 1). Thus, this review aims to describe how T. cruzi genetic diversity influences the biology of the parasite and/or clinical parameters in humans.
2 Epidemiology and DTU geographic distribution
Chagas disease is endemic to 21 countries and is considered a neglected disease by the WHO (51–53). In some regions, especially in countries in the Southern Cone of America, the acute phase of the disease had a clear reduction due to the success of vector and blood bank control. Owing to the migration of individuals with Chagas disease and the possibility of transmission by other routes, such as blood transfusion, congenital, and organ transplants in individuals chronically infected with T. cruzi (54, 55), the infection has also acquired importance in non-endemic areas of Chagas disease (56, 57).
An outstanding characteristic of T. cruzi is its significant plasticity, characterized by genetic variability and intense intraspecific phenotypic diversity. Regarding geographic dispersion, evident differences in T. cruzi DTU distribution were observed in distinct areas. For example, all DTUs were identified in South America, while TcI, TcII, and TcIV were identified in North America, and only TcI and TcIV were detected in the hosts and vectors from Central America (42, 49). TcI is the most ubiquitous DTU found in the wild cycle throughout all endemic areas. In the domestic cycle of the T. cruzi infection, it was widely prevalent in patients from North and Central America, Colombia, and Venezuela (7, 42, 58–60); some cases have also been identified in Chile and Argentina (21, 42, 61). The TcI has been frequently associated with outbreaks of oral transmission in the Northern Amazon (39, 62), but not in other South American regions, where TcII, TcV, and TcVI are more related to chronic infection, regardless of the clinical manifestation of Chagas disease (63–65).
TcII is the main DTU detected in Brazilian patients from the Central and Southeastern regions, where there were also registers of TcVI as a secondary DTU (66, 67). TcII is abundant in Chile and Colombia and less frequent in other regions (21, 42, 68).
TcIII and TcVI were predominantly associated with sylvatic cycles of the T. cruzi infection. TcIII was detected in sylvatic hosts from Northeastern Venezuela to Argentina, but infection in dogs and humans has been reported in Colombia (69, 70) and Argentinean Chaco (71–73). TcIV is a relevant agent in Chagas disease in Venezuela (58). It has also been reported in oral outbreaks in the Brazilian Amazon region (39, 62, 74).
TcV and TcVI were observed predominantly in the Southern Cone greater Gran Chaco (Peru, Bolivia, Northern Chile, and Argentina), Paraguay, and Uruguay. There are some registers of TcV in the extreme South of Brazil and Paraguay (5, 73). Some cases of human T. cruzi infection with TcV and/or TcVI have been reported further north in Ecuador (75) and Colombia (41, 76). In Bolivia, all DTUs were identified as circulating in humans except TcIII (42), with a predominance of TcI and TcV.
In addition, to the ecogeographic diversity, the literature demonstrated variations in biological and biochemical characteristics between T. cruzi subpopulations. In 1909, Chagas already described the morphological differences in the blood forms of the parasite. Generally, the stout forms of T. cruzi remain in the bloodstream and are unable to invade host cells rapidly, while the slender forms invade cells and start the intracellular cycle more quickly. The variation in parasitemia and tissue tropism correlates with the morphology of the T. cruzi forms because different strains have variable numbers of each form (77, 78). Miles et al. (1981) (58) described the first evidence that chronic Chagas disease manifestation could differ according to strain type and geographical location. In Venezuela, patients rarely develop mega-syndromes, while central and eastern Brazil have common cardiac and mega-syndrome manifestations (58). Although considerable advances have been made in the understanding of the physiopathogenesis of Chagas disease, no prognostic factors still allow a conclusive definition of patients infected with T. cruzi who develop symptomatic clinical forms. This multifactorial process involves host, parasite and environmental features, so a broad approach must be considered to better understand the factors that influence the physiopathogenesis of Chagas disease (79–81). Considering the T. cruzi genetic diversity, the current data corroborate Miles’ observations since the digestive forms are associated with infections where TcII, TcV, and TcIV DTUs were identified, while indeterminate forms and cardiac forms could be related to different DTUs with greater or lesser severity depending on the geographic regions (73, 82, 83). Differences in the capacity of T. cruzi for cell penetration, infectivity, differential induction of the immune response, tissue tropism, and response to treatment have already been demonstrated (84–86), which could confer differential capacity for adaptation to the host, influencing the transmission, response to the treatment, and the course of the infection (5, 87). Several studies have also demonstrated that distinct T. cruzi strains could trigger different immune responses and be influenced by geographical location (31, 88–93).
In addition, T. cruzi mixed infections and reinfections can alter the natural course of infection compared to individual populations, giving the parasite new properties and hampering correlations (13, 89, 94–96).
3 Infectivity and virulence of T. cruzi DTUs
The first criteria for subdivision of T. cruzi were based on parameters such as infectivity and virulence in mice infected with distinct parasite strains (12, 97). Andrade and Magalhães (1997) (97) characterized Type I as strains with a prevalence of thin forms and macrophage tropism that multiply quickly, developing high parasitemia and consequently leading to the death of Swiss mice in the first days after infections (7th–12th days). Type II T. cruzi strains have a frequency of wide forms and myocardial tropism that multiply slowly and present irregular peaks of parasitemia between the 12th and 20th day after infection. Moreover, the authors considered Type III as a T. cruzi strain with a prevalence of wide forms and skeletal musculature tropism, which multiply slowly and present late parasitemia peaks (20th–30th days), as well as low mortality rates in Swiss mice.
The correlation between the genetic variability of T. cruzi and its biological properties (60) has been scientifically sustained and corroborated in several studies with strains and clonal stocks of the parasite in vitro (98–100), experimental mouse infections (84, 100–102), and vectors (103–106). In general, these studies demonstrated that TcI presented higher multiplication and survival in acellular and cellular cultures than TcII when the biological behavior of clones (13, 98, 99) and strains of T. cruzi was evaluated (97, 107, 108). Revollo et al. (1998) (99) and Nogueira-Paiva et al. (2015) (108) used the “Vero” cells (cells isolated from African Green monkey kidney obtained from the Laboratory of Parasitology at the Pasteur Institute in Paris) to show differences in multiplication and survival when comparing the growth of different DTUs of T. cruzi in vitro, whereas Andrade and Magalhães (1997) (97), Rimoldi et al. (2012) (107) and Andrade et al. (2010) (13) used different mice organs and cells to demonstrate the distinct biological behavior of T. cruzi genotypes. Oliveira et al. (2017) (100) showed different results regarding the biological behavior in vitro of parasites isolated from patients with chronic Chagas disease. These authors verified that one sample of TcII demonstrated more significant growth in acellular culture than TcI and TcVI, and two other samples of TcII showed similar growth. The authors also revealed that the infectivity of “Vero” cells after 72 h was similar for all DTUs. However, one sample of TcII DTU yielded a higher number of amastigotes per cell.
The genetic variability of T. cruzi can influence parasite infectivity, reproduction, and differentiation in vectors (103, 104, 109, 110). Previous studies have demonstrated that clones of TcI are better able to infect and complete the life cycle in the Triatoma infestans vector than TcII clones, indicating that the DTUs do not exhibit the same vectorial transmissibility (103, 104). De Abreu et al. (2022) (111) showed differences in vector susceptibility to infection and competence when two vector species were infected with TcI, TcII, and TcIV strains from Amazon (AM) and Paraná (PR) in single and mixed T. cruzi infections. It is verified that Rhodnius robustus showed vector competence for TcIV (AM) and TcI (AM) + TcIV (AM), and Rhodnius pictipes showed vector competence for TcI (AM) + TcIV (AM) and TcI (AM) + TcII (PR).
The biological behavior of T. cruzi DTUs such as parasitological parameters, infectivity, and virulence was also assayed in the vertebrate hosts (84, 90, 91, 97, 100, 112–121). Lana et al. (2000) (113) revealed that 19/20 (TcI), 32 (TcII), and 39 (TcV) clonal T. cruzi genotypes exhibited high, intermediate, and low maximum parasitemia peaks, respectively, indicating that the clonal genotypes differed significantly in their infectivity in BALB/c mice. Toledo et al. (2002) (84) verified the biological behavior of a broader panel of T. cruzi clonal stocks. They demonstrated differences in parasitemia, infectivity, mortality, tissue parasitism, and inflammatory processes during the acute and chronic phases of experimental T. cruzi infection in BALB/c mice. The authors concluded that closely related clonal T. cruzi genotypes, 19 vs. 20 (TcI) and 32 (TcII) vs. 39 (TcV), showed, in general, fewer differences among themselves than distantly related groups, 19 or 20 (TcI) vs. 32 (TcII) or 39 (TcV). Some studies have shown that TcI has a high ability to infect experimental BALB/c (85) and Swiss mice (97, 112), developing larger parasitemia than other T. cruzi genetic groups (85, 97, 112). However, it has been demonstrated that samples of TcI isolated from patients with chronic Chagas disease may also present low levels of parasitemia in Swiss mice (100, 118). Sales-Campos et al. (2015) (121) verified that strains belonging to TcI exhibited low levels of parasitemia in BALB/c mice. T. cruzi stocks belonging to TcI and TcIV from the Brazilian Amazon diverged in terms of biological and medical properties in Swiss mice, while TcIV displayed higher virulence and parasitemia parameter values, and TcI determined the most severe inflammatory process and higher frequency of tissue parasitism (117). These infectivity and virulence alterations in TcI may be associated with intra-DTU differences. In contrast, TcII isolated from T. cruzi-infected humans exhibits high infectivity and virulence in experimental Swiss mice (90, 100, 114, 116). Lisboa et al. (2007) (122) studied sylvatic T. cruzi stocks and verified higher parasitemia in Swiss mice infected with TcII than with TcI. Significant biological heterogeneity was also observed in T. cruzi TcII isolates derived from L. rosalia from the Atlantic Forest, unlike the TcII isolates derived from marsupials, which present similar profiles. Together, these data confirm the high level of intraspecific divergence in T. cruzi and that the behavior of isolates can vary according to geographic and host origin (85, 117, 122, 123).
Standard T. cruzi strains, representative of the major genotypes of the parasite, have also been used to evaluate the biological behavior of DTUs in experimental animals (91, 124). Magalhães et al. (2015) (119) showed that Colombiana (TcI) and Y (TcII) strains lead to the differential activation of human monocytes and T cells, which might influence disease progression. Corroborating this work, Duz et al. (2014) (91) indicated that dogs infected with the Colombiana strain (TcI) reached the parasitemia peak later than animals infected with the Y strain (TcII) and that the Y strain (TcII) triggered a more intense immune response during the acute phase of infection in dogs. This is consistent with the Colombiana strain (TcI) in experimental models that induced a milder infection than the Y strain (TcII).
Studies using other strains isolated from T. cruzi DTUs, such as TcIII, TcV, and TcVI, have also verified the infectivity and virulence of these genetic groups in C57BL/6J mice (115, 120). Ragone et al. (2015) (120) showed that TcVI induces high parasitemia, severe histological damage in the heart, and moderate lesions in C57BL/6J mice skeletal muscle. On the other hand, TcIII presents less parasitemia than TcVI and mild-to-moderate histological damage in the heart and skeletal muscle. Lastly, TcV induced subpatent parasitemia and mild lesions in the analyzed tissues.
In natural infections with T. cruzi, it is crucial to consider that mixed infections with multiple DTUs are common and can lead to changes in the biological properties of the parasite as well as the clinical course of Chagas disease (94, 95, 105, 113, 125–127). Some studies have demonstrated that T. cruzi clones with less ability to develop in invertebrate and vertebrate hosts during monoclonal infections can multiply in mixed infections (94, 104, 105, 113, 128). It has been demonstrated in vivo that the tissue tropism of one T. cruzi DTU could change in the presence of other DTUs (124, 129). Moreover, the histopathological damage and intensity of the inflammatory process resulting from these mixed T. cruzi infections also present remarkable variations (89, 130).
4 Immune response and cell interaction
The immune response against Chagas disease has been investigated for many years and can generate protective or pathogenic events. The host’s genetic background, in addition to the specific characteristics of the parasite, seems to play a relevant role in influencing the outcome of the disease (131). The murine model of Chagas disease has been well established and effectively reflects the key features of human T. cruzi infection. Notably, mice’s immune response and cytokine expression levels during the acute and chronic phases closely resemble those observed in human Chagas disease (132). Ferreira et al. (2018) (132) assessed aspects of the acute and chronic phases of T. cruzi infection using G (TcI) and CL (TcVI) strains in two distinct mouse lineages (C57BL/6 and BALB/c). These findings revealed that the CL strain established infection more rapidly than the G strain. Concurrently, the immune response in BALB/c mice was initiated earlier than that in C57BL/6 mice. In the acute phase, all animals responded to T. cruzi infection by elevated serum concentrations of cytokines, with BALB/c mice demonstrating a more regulated immune response than C57BL/6 mice. In the chronic phase, C57BL/6 mice continued to exhibit exacerbated cytokine and chemokine responses. Despite differences in T. cruzi tropism among parasite strains, host background can significantly influence immune responses throughout infection (132).
Previous studies have demonstrated variations in the features of T. cruzi infection based on the mouse lineage and parasite strain used in the infection (133–135). In a study conducted by Sanoja et al. (2013) (134), a comparative analysis of CD4+ T-cell subset dynamics was performed after infection with the Y strain during the acute and chronic phases in BALB/c and C57BL/6 mice. The findings revealed that C57BL/6 mice exhibited heightened levels of CD4+ T-cell infiltration and expression of Th1 cytokines in the heart, coupled with the presence of Treg cells. In contrast, BALB/c mice showed a higher heart parasite burden, lower heart CD4+ T-cell infiltration, and reduced levels of Th1 and inflammatory cytokines, but with an increased presence of Th17 cells. In the chronic phase, BALB/c mice continued to exhibit higher parasite burdens than C57BL/6 mice, along with elevated levels of interferon (IFN)-γ, tumor necrosis factor (TNF), interleukin (IL)-10, and transforming growth factor (TGF)-β (134). Domingues et al. (2020) (135) also explored the influence of host genetics on histopathological and immunological aspects following infection with the SC2005 T. cruzi strain (isolated from a patient with Chagas disease) in BALB/c and A mice. They observed that BALB/c mice displayed higher parasitemia and mortality rates than A mice. Despite both lineages demonstrating a resistant immune profile, characterized by an increase in CD8+ T cells in the heart, liver, and blood; an increase in CD19+ B cells in the liver; and high levels of proinflammatory cytokines, the response to infection occurred later in BALB/c mice. Consequently, A mice were less susceptible to T. cruzi infection. The host genetic background can influence the early development of a cytotoxic cellular response profile, crucial in developing a less severe manifestation of Chagas disease (135). Other studies also support the significance of host genetic background in the clinical manifestations of chronic Chagas disease (136, 137). Frade et al. (2013) (136) analyzed CCR5, CCL2, and MAL/TIRAP genes in patients with chronic chagasic cardiomyopathy. Their findings demonstrated that the CCL2rs2530797A/A and TIRAPrs8177376A/A genotypes were associated with increased susceptibility, whereas the CCR5rs3176763C/C genotype was associated with cardiomyopathy protection (136).
The entry of T. cruzi into cells is a multifactorial process (138). Recent studies have indicated that many molecules interacting with specific cell receptors are expressed on the surface of T. cruzi, allowing the invasion of several cell types and the modulation of cell metabolism to enable its survival (139). Among the proteins involved in this process are GPI-anchored mucins, glycophosphatidylinositol, transialidase, and cruzipain, which are involved in the differential capacity of cell adhesion of T. cruzi strains and evasion of the immune response (140, 141).
The expression levels and glycosylation pattern diversity of mucin-associated surface proteins (MASPs) can vary among different T. cruzi strains, influencing the parasite’s virulence and immune response. MASP is the second-largest multigene family in T. cruzi and comprises approximately 1,300 genes. MASP proteins are characterized by a highly variable and repetitive central region comprising peptides shared among all MASP members. The pronounced polymorphism within this family and its surface location on infective forms of T. cruzi imply that MASP actively participates in host–parasite interaction mechanisms. Initial proteomic findings related to the MASP family suggested potentially low expression levels, with only four proteins identified in the trypomastigote stage and one in the epimastigote stage. One hypothesis posits that MASPs may be predominantly expressed during the intermediate stages of the parasite’s life cycle or undergo post-translational modifications, such as distinct N-glycosylation (142). Subsequent research on these genes has revealed protein expression in epimastigotes, trypomastigotes, and amastigotes of T. cruzi. Moreover, MASPs could be secreted during the early stages of amastigote genesis in vitro at pH 5.0 (143, 144). The expression profiles of these proteins may vary throughout infection, with different strains exhibiting differential expression of various MASP family members (145). Notably, immature MASP proteins and their C-terminal portion have been identified in trypomastigotes’ exosomes, showing immunogenic properties (146, 147). Recent studies have demonstrated that host antibodies against MASPs may exhibit strain-specific recognition patterns. Understanding the variation in surface-expressed molecules and diversity of MASPs among different T. cruzi strains is crucial for unraveling the complexity of Chagas disease and developing targeted interventions, including vaccines and treatments. A few years ago, a synthetic peptide derived from T. cruzi MASP was tested as a vaccine candidate against Chagas disease (148). This vaccine candidate induced an 86% survival rate in the immunized group after challenge with a highly lethal dose of trypomastigotes. Immunized animals also exhibited the lowest parasite load in the heart, liver, and spleen compared with control animals, as demonstrated by real-time polymerase chain reaction (PCR) (148). Ongoing research endeavors continue to unveil the intricacies of T. cruzi diversity and its implications in disease pathogenesis (148).
Gp90 has been described as a molecule with an antiphagocytic effect in mammalian stages of T. cruzi (149) and is considered a negative modulator of infection (150). Gp82 and gp35/50 are also implicated in cell invasion and are expressed on the surface of metacyclic trypomastigotes of T. cruzi. The mucin-like gp35/50 is resistant to protease digestion and responsible for protecting the parasite from destruction by the oral route (150). Gp83 is a ligand employed by the parasite to attach to and enter phagocytic and non-phagocytic cells (140, 151). All were expressed on the surface of metacyclic trypomastigotes.
T. cruzi has been reported to activate Toll-like receptor 2 (TLR2), TLR4, and TLR9 (152, 153), and this innate recognition mechanism can influence cell activation and infectivity (Figure 1). TLRs are crucial components of the innate immune system, recognizing various pathogen-associated molecular patterns (PAMPs) and initiating immune responses (152–156). These receptors are important transmembrane proteins that confer a certain degree of specificity to the innate immune system cells. This innate recognition mechanism can influence cell activation and infectivity (Figure 1). Each TLR initiates downstream signaling that culminates in activating signaling pathways that regulate the expression of cytokines, chemokines, and interferons (153). Cell surface TLRs mainly recognize microbial membrane components such as lipids, lipoproteins, and proteins; intracellular TLRs recognize nucleic acids derived from bacteria and viruses and self-nucleic acids in disease conditions such as autoimmunity (155). TLR2, TLR4, and TLR9 are specifically mentioned in the context of T. cruzi infection. TLR2 recognizes various PAMPs, including lipoproteins and glycolipids (152–154, 156, 157). Studies have shown that TLR2 can be activated by parasite-derived molecules, contributing to the host immune response (153). TLR4 is primarily associated with bacterial infections for recognizing lipopolysaccharide (LPS), and some studies suggest its involvement in parasitic infections as well. The activation may occur through the recognition of parasite components (153). TLR9 recognizes bacterial and viral DNA that is rich in unmethylated CpG-DNA motifs; in the case of T. cruzi, the activation may be related to the presence of parasitic DNA, and additional studies attributed the production of proinflammatory cytokines (TNF and IL-12) to the cooperative activation of TLR2 and TLR9 (152, 157). This recognition can lead to the induction of pro-inflammatory responses and the initiation of an effective immune reaction against the parasite.
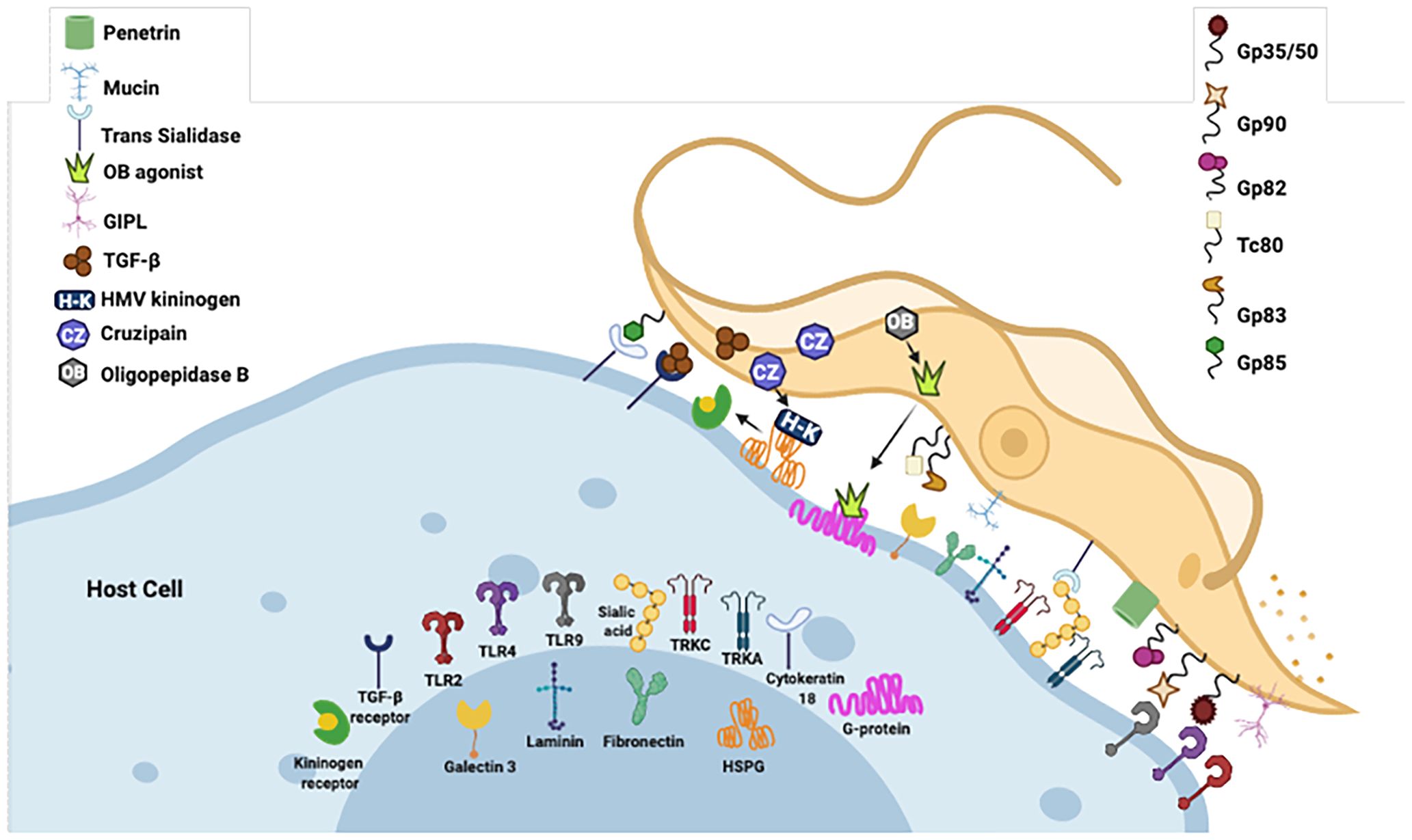
Figure 1 Examples of molecules expressed on the surface of T. cruzi that interact with specific cell receptors. Gp, glycoprotein; TLR, Toll-type receptors; MVs, microvesicles; LPS, lipopolysaccharide; PAMP, pathogen-associated molecular patterns; ssDNA, single-stranded DNA. Figure created by the author on the biorender.com platform, inspired by an Oswaldo Cruz Foundation website publication.
Although the adaptive immune response has been considered for many years to be the most relevant in building the balance between protective and pathogenic events during chronic infection of Chagas disease, several studies have demonstrated the importance of the innate immune response as a significant factor in this issue, mainly in the process (154). During the acute phase, cells of the innate immune system play a pivotal role in controlling the infection by suppressing parasite replication. The classic mechanism proposed is that T. cruzi initiates a cascade of events that trigger the synthesis of IL-12 by macrophages, which is a crucial mediator of IFN-γ production through Th1 and NK activation (158, 159) (Figure 2). IFN-γ plays a crucial role during T. cruzi infection by increasing the production of IL-12, TNF-α, and nitric oxide (NO) by macrophages. The NO is cytotoxic to intracellular microorganisms and exerts trypanocidal activity, inhibiting the development of T. cruzi (160–162) (Figure 2). Moreover, IFN-γ stimulates T-cytotoxic lymphocyte activation, a central mechanism for systemic and antigen-specific protection against T. cruzi intracellular infection (163, 164) (Figure 2).
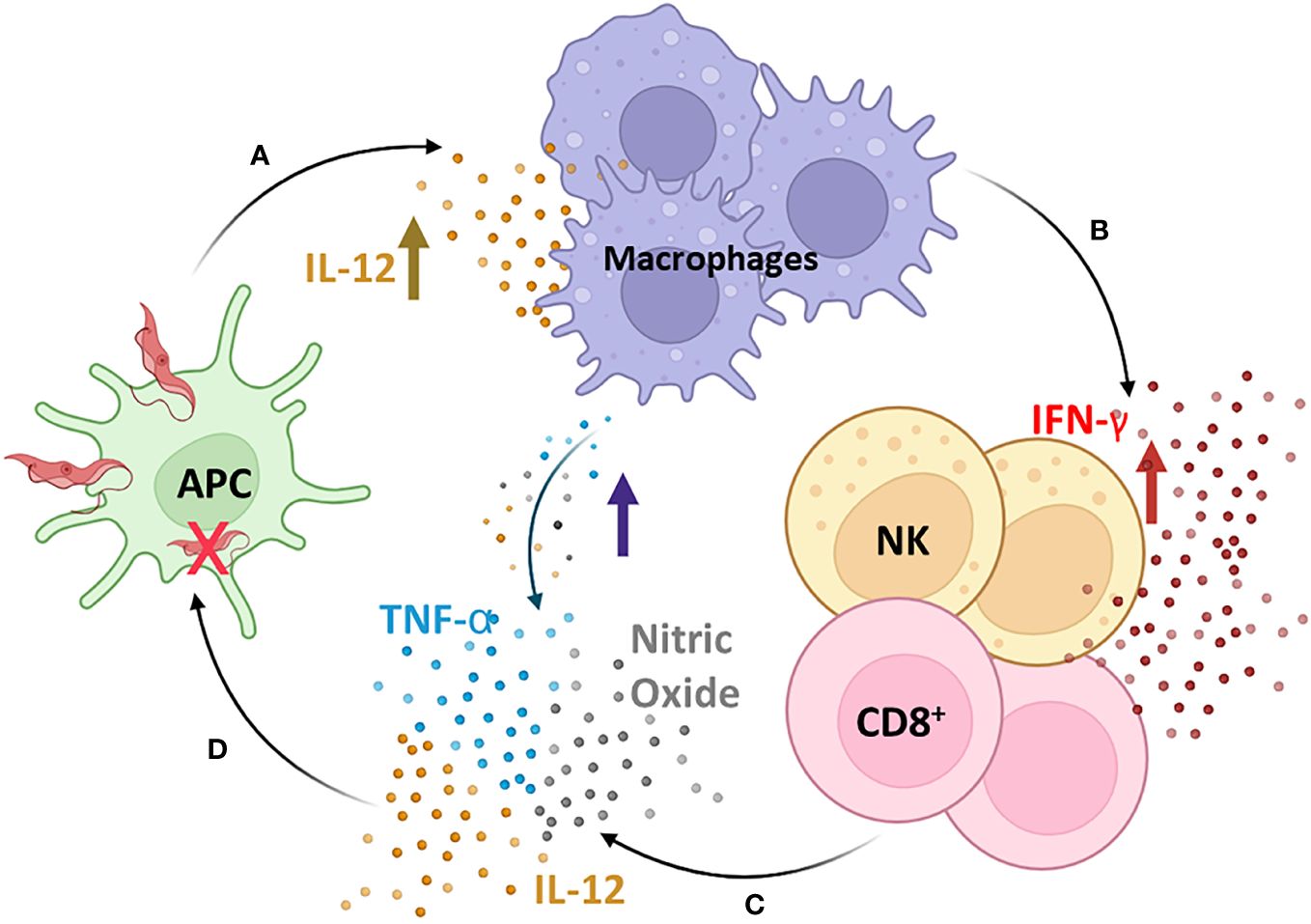
Figure 2 The cascade of induction of the innate immune response. T. cruzi initiates a cascade of events that trigger the synthesis of IL-12 by macrophages (A), which is a crucial mediator of IFN-γ production through Th1 and Natural Killer (NK) activation (B). IFN-γ plays a crucial role during T. cruzi infection by increasing the production of IL-12, TNF-α, and nitric oxide (NO) by macrophages (C), which are cytotoxic to intracellular microorganisms and exert trypanocidal activity, inhibiting the development of T. cruzi (D). Moreover, IFN-γ stimulates T-cytotoxic lymphocyte activation, a central mechanism for systemic and antigen-specific protection against T. cruzi intracellular infection. APC, antigen-presenting cells; NK, natural killer cells; CD8+, CD8+ T lymphocytes. Figure created by the author on the biorender.com platform, inspired by an Oswaldo Cruz Foundation website publication.
One of the first lines of defense is the complement system, which consists of more than 35 plasma and cell surface receptors/regulators. After interaction with the pathogen, it can be activated by three pathways of the protease cascade: classical (CP), alternative (AP), and lectin (LP), resulting in inflammation, opsonization (phagocytosis), and lysis of pathogens (Figure 3) (165–167). Although these pathways differ in the initial steps of their respective cascades, all three converge to produce a C3 convertase and then a C5 convertase, forming a membrane attack complex (MAC) and subsequent pathogen lysis (167). The main mechanisms involved in the ability and capacity of trypomastigotes to avoid complement action are as follows: T. cruzi calreticulin (TcCRT), which is a 45-kDa calcium-binding protein, primarily located in the endoplasmic reticulum (ER) and expressed in infective trypomastigotes. After infection, TcCRT translocates to the emerging area of the flagellum on the plasma membrane surface (168–170). This protein can bind to host mannose-binding lectin (MBL) and interacts with ficolins, preventing C4 conversion to C4b and C1q and interfering in the activation of the CP and LP (170–172). TcCRT has also been shown to internalize parasites into mammalian cells (171). Another mechanism is T. cruzi Complement Regulatory Protein (TcCRP): the protein TcCRP, also called Gp160, is a 160-kDa glycoprotein anchored into trypomastigote membranes that can bind to C3b and C4b, preventing the assembly of proteolytically active C3 convertase, thus inhibiting the formation of CP and AP complement C3 convertase (168, 170, 173). The T. cruzi complement C2 receptor inhibition trispanning (TcCRIT) is a 32-kDa transmembrane protein mainly expressed in trypomastigotes. TcCRIT has amino acid sequence homology with the C4 beta-chain, the binding site of C2. Thus, it blocks C2 cleavage by C1s or MASP-2 into C2a and prevents C3 convertase formation, thus regulating the activation of the LP and CP (174–177) mediating cell lysis, allowing survival and cell invasion by the parasite. The glycoprotein 58/68 (Gp58/68) is a glycoprotein of 58 kDa (non-reduced) and 68 kDa (reduced) (178), expressed on the parasite surface or released by trypomastigotes. It is a part of the fibronectin/collagen receptor of T. cruzi and plays an important role in the interaction of T. cruzi with mammalian cells (178, 179). This protein was able to inhibit the formation of cell-bound and fluid-phase C3 convertases (178) to allow the parasite to evade AP complement activation by preventing the initial association of factor B (FB) with surface-fixed C3b (167, 178). The trypomastigote decay-accelerating factor (T-DAF) is an 87- to 93-kDa glycoprotein expressed on the surface of metacyclic trypomastigotes of T. cruzi. T-DAF mimics the activity of the complement regulatory protein DAF and regulates the activation of the AP, CP, and probably the LP of the complement by interfering with the assembly efficiency of C3 convertases (180, 181), which is essential for the escape of T. cruzi from complement activation and lytic effects (167).
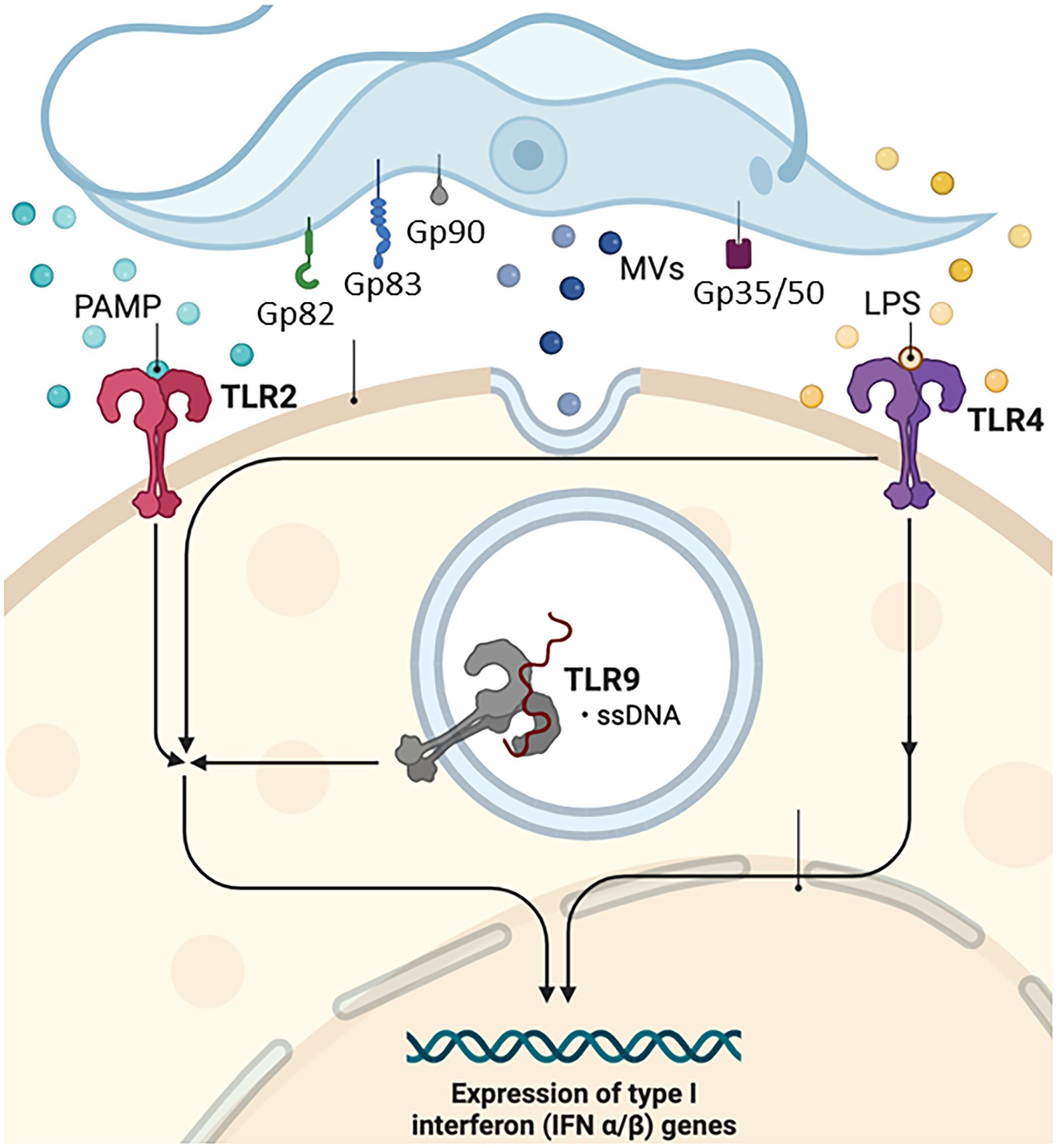
Figure 3 Complement system. Gp, glycoprotein; Tc, T. cruzi molecule; TLR, Toll-type receptors; MAC, complement membrane attack complex; MBL, mannose-binding lectin; MVs, microvesicles; T-DAF, Trypanosoma-decay accelerating factor. Figure created by the author on the biorender.com platform, inspired by an Oswaldo Cruz Foundation website publication.
Extracellular vesicles, whether microvesicles (MVs) or exosomes, shed by pathogens, transfer virulence factors and biomolecules to host cells, thereby altering the host’s susceptibility to infection (177) (Figure 1). Cestari et al. (2012) (176) and Wyllie and Ramirez (2017) (177) described a mechanism of complement immune evasion by T. cruzi through the induction of membrane-derived vesicles from host cells by metacyclic trypomastigote forms and the consequent secretion of these MVs. MVs are 100- to 1,000-nm molecules produced by outward budding and fission of the plasma membrane, released by cell blood, immune system, epithelial, and endothelial tissues, among others. They are an integral part of the intracellular microenvironment and act as regulators of cell-to-cell communication (182, 183). The complement system is a fundamental immune response component, recognizing and eliminating pathogens. T. cruzi may evade immediate immune responses by inhibiting the complement system, promoting its survival in the host environment (177). The infective form of the parasite induces the release of MVs from immune cells in a calcium-dependent manner at the beginning of infection. These MVs bind LP (177) and CP C3-convertase complexes on the surface of T. cruzi inhibiting complement-mediated lysis and favoring invasion and infection of host cells (176). Interestingly, MVs derived from different parasite strains (TcI and TcII) did not alter complement resistance and the invasion process (177). These studies provide valuable insights into the complex interplay between the parasite and host cell-derived MVs, establish a communication network that allows it to manipulate host cell functions, modulate immune responses, and potentially escape immune surveillance, contributing to the chronicity of T. cruzi infection and the establishment of persistent parasitemia (176).
According to Quijano-Hernandez and Dumonteil (2011) (184), the protective immune response against T. cruzi requires the activation of a Th1 immune profile with stimulation of T-cytotoxic cells and B-lymphocytes to secrete parasite-specific immunoglobulins (185). Furthermore, the control of parasite infection spread has also been associated with NK cells (186). Sathler-Avelar et al. (2006) (187) suggested that NK cells could protect against early T. cruzi infection, comparing the cytokine profile of patients with Chagas disease with healthy uninfected children. Vitelli-Avelar et al. (2005) (188) analyzed the frequency of NK cells in the peripheral blood of patients with Chagas disease, both of which were particularly high in indeterminate patients. These authors suggested that increased circulating NK cells may protect the host from morbidity during the chronic phase.
It has been proposed that T. cruzi DTU promotes diverse host immune responses and affects disease progression (Table 1). Similarly, anti-inflammatory and pro-inflammatory cytokines may play a pivotal role during infection and the differential regulation of cytokine synthesis in developing the Chagas cardiomyopathy or indeterminate clinical status (31, 188–190). It has been described that the lower expression of IL-10 is associated with the development of chagasic cardiomyopathy (190), and a higher expression was observed in patients with the indeterminate form (188–191), suggesting that IL-10 contributes significantly to parasite control without severe damage, regulating immune response during chronic Chagas disease. Magalhães et al. (2015) (119) showed that while TcI DTU induced IL-10 production and higher monocyte activation, TcII DTU led to lower monocyte activation but a higher inflammatory profile. TGF-β was demonstrated to play a role in regulation during the acute phase of Chagas disease, whereas it was shown to be a potent inhibitor of the effects of macrophage-activating cytokines, such as IFN-γ (192). IFN-γ and TNF-α, inflammatory cytokines, and cytotoxic cells have been correlated with tissue damage and the severity of chronic Chagas disease (190, 193–195) in cardiac patients, suggesting a role for pro-inflammatory monocytes in developing this disease. Guedes et al. (2012) (35) showed that higher levels of IL-17 were associated with less aggressive chagasic cardiomyopathy.
Distinct T. cruzi DTUs may exhibit variations in parasitemia, immune responses, and tissue pathology in their hosts (89–91, 93). Moreover, these parameters may differ in co-infections compared to T. cruzi mono-infections (89). In a study comparing the biological behavior of TcII and TcVI DTUs conducted by Rodrigues et al. (2010) (89), it was demonstrated that TcII presented reduced parasitemia in mice compared to TcVI. TcVI induced higher parasitemia, increased the systemic release of pro-inflammatory mediators, and induced higher mortality during the acute phase of the disease. Co-infection with TcII and TcVI results in intermediate parasitemia, heart parasitism, and mortality. This finding correlated with a decrease in inflammatory cells, suggesting that the T. cruzi co-infected animals exhibited a less intense inflammatory reaction in the heart. These data indicate that T. cruzi co-infection can trigger both protective inflammatory immunity and regulatory immune mechanisms that attenuate the damage caused by inflammation, consequently reducing disease severity (89). However, when the biological behaviors of TcII and TcIV were compared in infected mice, TcIV showed lower virulence and fewer inflammatory processes than TcII. This includes significantly lower parasitemia levels and a lower frequency of organs with inflammatory processes (90). Magalhães et al. (2019) (93) observed that TcIV induced a regulated profile in human monocytes, whereas TcV induced an inflammatory profile. Differences in the host’s cellular immune response were noted considering the different DTUs and phases of T. cruzi infection (91). In an experimental model using dogs, Duz et al. (2014) (91) proposed that, in the acute phase, TcI could go unnoticed by peripheral blood mononuclear cells, enabling faster parasitization of target organs. In the chronic phase, TcI presents characteristics of inflammation, with higher levels of IL-4 observed in the peripheral blood. TcII DTU triggered the production of IFN-γ and IL-4 in the acute phase, and significant heart inflammation and fibrosis were observed in the chronic phase. Differences in humoral immune response were also associated with different DTUs during acute and chronic T. cruzi infection in mice. TcI is more efficient in overexpressing specific antiparasitic IgG subclasses (IgG, IgG1, IgG2a, and IgG2b) than TcII and TcV (88). This finding was recently corroborated by Silveira-Lemos et al. (2021) (81), who demonstrated that distinct T. cruzi genotypes influence the phenotypic and functional features of the host immune response.
The host genetic background plays an important role in the course of infection, such as the genetic variability among the six different DTUs of T. cruzi that interact differently with the host and their eco-epidemiological effects. The immune response is decisive throughout the entire process. The cytokine profiles switch between anti-inflammatory and pro-inflammatory cytokines, which may be relevant in determining chronic patients’ clinical presentation and disease outcomes. These results show that the progression of human Chagas disease from asymptomatic to severe forms is related to a lack of adequate immune modulation.
5 Pathogenesis and DTU
The clinical course of Chagas disease is polymorphic, ranging from asymptomatic to severe chronic cardiovascular or gastrointestinal involvement. After parasite transmission, trypomastigote forms of T. cruzi can invade different cell types, including macrophages, muscle cells, and fibroblasts. Parasitic amplification into the cytosol results in intense tissue parasitism and blood parasitemia during the acute phase. The symptoms in this phase are generally mild, except in immunosuppressed patients and children who may develop cardiomyopathy and encephalomyelitis (196, 197). After the acute phase, parasitemia decreases in the chronic phase of the disease, starting with the asymptomatic or indeterminate form, where no clinical symptoms are observed. Most individuals remain in this phase of infection throughout the rest of their lives. Approximately 30% of these patients develop chronic phase disease with cardiac and/or gastrointestinal symptoms. This involvement may be severe and a determinant of the morbidity of the disease (32, 198, 199).
Cardiomyopathy is one of the most important clinical changes in patients with Chagas disease in the chronic phase due to the high frequency with which it develops (in 20%–30% of infected individuals), in addition to its severity, morbidity, and mortality (200). The classic pathology of chronic Chagas cardiomyopathy includes myocarditis accompanied by myocytolysis, myofiber hypertrophy, and interstitial fibrosis (201). In general, only focal areas of inflammation are found in the hearts of patients with chronic Chagas disease. Inflammatory infiltrates mainly comprise T cells, macrophages, eosinophils, plasma cells, and mast cells (200). Fibrosis in chronic Chagas disease exhibits a unique distribution pattern, often surrounding and involving individual myocardial fibers. This feature distinguishes it from the fibrosis patterns in idiopathic dilated cardiomyopathy (202, 203). Moreover, fibrosis in Chagas disease can also be characterized by intrafascicular deposition of collagen (204, 205), which causes disorganization and isolation of cardiomyocytes and contributes to the electrocardiographic changes observed in a canine model and in humans (206). The host innate and adaptive immune responses may contribute to cardiac damage and increase the risk of heart failure through the induction of inflammation, fibrosis, and oxidative stress injury, leading to myofibril rupture, cardiomyocyte necrosis, microvascular dysfunction, autonomic dysfunction, cardiac hypertrophy, and fibrosis (200). Duz et al. (2014) (91) evaluated the immune response and cardiac lesions in dogs infected with Colombian (TcI) and Y (TcII) strains of T. cruzi. The authors demonstrated that in the chronic phase, the inflammation triggered by the Y strain was balanced by tissue rearrangement and fibrosis, whereas infection with the Colombian strain showed more significant inflammation and a lower degree of cardiac fibrosis. Ferrer et al. (2014) (207) analyzed the impact of different T. cruzi isolates obtained from Argentine patients (TcI, TcV, or TcVI) on cardiac tissue from T. cruzi-infected CF1 mice and showed that the degree of myocarditis ranged from minimal to mild for TcV and TcVI to moderate to intense for TcI. T. cruzi DTUs define the pattern of inflammatory mediators in heart tissue and may contribute to the magnitude of cardiac pathogenesis (208).
Controversies surround the question of the relative contribution of autoimmunity and the presence of the parasite to the development of chronic T. cruzi infection. Persistent inflammation and divergence between low parasite load in the tissue and the severity of the lesions observed during the chronic phase support this autoimmune etiology (209, 210). The autoimmunity hypothesis suggests that tissue damage leads to an immune reaction against self-proteins. This theory has been considered a key mechanism to explain the tissue damage observed in the chronic phase of the Chagas disease, even in the absence of parasites in the affected tissues.
However, despite the wide acceptance of autoimmunity in the etiology of Chagas disease, several studies have suggested a strong association between parasite load and disease severity (211–214). Even if anti-self-immune responses are induced in these models of Chagas disease, these responses are insufficient to generate disease without local parasitic infection. The direct pathogenic role of the parasite has gained strong support from very sensitive new immunohistochemical techniques and applications of PCR, which show a strict correlation between the presence of the parasite and tissue lesions (210, 215, 216).
The circulation of distinct genotypes of T. cruzi within infected triatomines suggests complex interactions among various parasites, leading to diverse infection patterns (125, 217). This genetic diversity may account for regional variations in the incidence of the cardiac and/or digestive forms of the disease. However, the distinct clinical manifestations of Chagas disease can arise from factors beyond the genetic diversity of T. cruzi, such as the interaction between the parasite and the host (60, 125, 190, 218).
The varied symptoms of Chagas disease likely result from differences in the immune response among individuals, which effectively control parasitic levels and limit organ damage, while inefficient responses fail to control parasite proliferation, resulting in persistent inflammation and severe clinical manifestations (219). Parasites selectively form multiclonal populations in different individuals, giving rise to stable lineages. According to the “clonal histiotrophic model” of Chagas disease, the polymorphism of biological factors allows distinct T. cruzi clones within a lineage to exhibit tropism for various tissues, which also contributes significantly to the clinical outcome of the disease (220, 221). Classic studies have demonstrated that the Y strain (TcII) is associated with high parasitism of the spleen, liver, and bone marrow in addition to increased virulence in the acute phase of the infection, whereas the Colombian strain (TcI) presents cardiomyotropism and pathogenicity in the chronic phase (78, 222, 223). The T. cruzi clone Sylvio-X10/4, belonging to the TcI DTU, also produces myocarditis in C3H/He mice, similar to that observed in human chronic Chagas disease caused by the Colombian strain (TcI) (224). On the other hand, different groups have pointed out that the Y strain (TcII) produces inflammation at the cardiac level in mouse models (225), and that infection with the CL (TcVI) strain results in high parasitism of countless cell types, indicating that the T. cruzi strain is pan-infective and does not affect only the heart tissue (226). In a canine model, the Y strain (TcII) triggers a more robust immune response during the acute phase of infection than the Colombian strain (TcI) (127). Colombian infection (TcI) shows characteristics of inflammation during the chronic phase. It is possible that this strain can escape the host’s acute immune response and parasitize the organs faster, while the Y strain (TcII) triggers a specific immune response during the acute phase, helping to control myocardial lesions in the early chronic phase (91). A more recent study realized by Reis Machado et al. (2014) (92) shows that the animals infected or reinfected with the Colombian strain (TcI) had a significantly higher expression of IFN-γ, both in the cardiac tissue and in spleen cells when compared to groups infected with the Y strain (TcII).
De Diego et al. (1998) (227) found qualitative and quantitative histopathological differences in studying T. cruzi genotypes belonging to different DTUs. Genotype 39 (TcV) was detected in infected mice’s skeletal muscle, spleen, liver, and heart. In genotype 20 (TcI), heart inflammatory foci were small. In genotype 19 (TcI), skeletal and cardiac muscle fibers were the most parasitized, demonstrating that differences can occur between T. cruzi strains in the same subgroup (206). In rats, infection with JG (TcII) and CL Brener (TcVI) revealed differential tissue tropism among the strains. While the JG strain (TcII) occurs in the cardiac muscle, CL-Brener (TcVI) is detectable in skeletal muscles and other organs (129). In a study of Colombian patients, TcII was detected in the heart, causing chronic chagasic cardiomyopathy (CCC). In contrast, TcI was found in the muscular layer of the esophageal tissue, along with lymphocytic infiltrates and interstitial fibrosis (126). In D’Avila et al. (2009) (228), the authors did not find a correlation between the genetic profiles of T. cruzi isolates and different clinical forms of Chagas disease. Likewise, Del Puerto et al. (2010) (64) analyzed blood samples from 306 Bolivian patients with Chagas disease in the chronic phase and found no associations between T. cruzi DTUs and the clinical manifestation of the Chagas disease. The differences between the findings indicate that histopathological studies can produce different results according to genetic variations between T. cruzi strains and the experimental model or type of clinical study performed.
It is well known that T. cruzi can change virulence and tissular tropism over time to maintain its infectious properties, depending on factors from the host (229, 230). Many groups have not successfully correlated the parasite’s genetic variability with the disease’s clinical characteristics since most studies on T. cruzi isolates and the parasite’s growth in laboratory animals or in vitro cultures have a high chance of clonal selection. Often, parasite populations in the heart, skeletal muscle, liver, or spleen differ from those found in the host’s blood (231). The presence of different T. cruzi DTUs with different tropisms in the same host originated from distinct populations of parasites in diverse tissues, even when infected with a particular strain. Some studies have demonstrated different T. cruzi genetic groups in the bloodstream and heart tissue of the same host (221, 232–234). These findings indicate the need to carry out studies with tissue and bloodstream samples from patients with Chagas disease, to compare all T. cruzi genotypes present in the same individual, as they will probably not be the same.
Differential tissue distribution of T. cruzi populations has been detected in male BALB/c mice infected with two clonal stocks (124). In short, using a quantitative assay to estimate the proportion of each clone in the profiles, 80% predominance of the strain Col.1.7G2 (TcI) concerning the strain JG (TcII) was observed in the rectum, diaphragm, esophagus, and blood of the infected mice, in contrast with up to 90% preponderance of the strain JG (TcII) in the heart of the same mice. Curiously, the strain Col.1.7G2 (TcI) used in this study was obtained from a patient with cardiac Chagas disease and showed tropism for smooth muscles in mice, while strain JG (TcII), obtained from a patient with the digestive form of the disease showed cardiotropism. Hence, the behavior of parasites within disparate reservoirs may exhibit variations (124, 232). Vago et al. (2000) (125) showed that parasites possessing distinct genetic profiles can be discerned in separate tissues, such as the esophagus and heart, within the same patient.
Studies that used an initial inoculum containing strains of different T. cruzi DTUs have detected the predominance of one strain over the others (120, 124, 127, 235, 236). The survival of only one isolate in a given tissue could be related to its ability to escape from the immune system or to a selective process within host cells (127). Thus, the distribution of different parasites in host organs could be influenced by the interactions between parasites of different groups, and the presence of a specific population of T. cruzi in tissues could be related to distinct clinical manifestations of the disease. The diversity of parasites triggers a more complex immune response that restricts the more susceptible parasites to specific organs or even removes them from the host. A particular population of T. cruzi could infect different tissues within the host, but their presence within a particular organ would be determined by other parasites in the co-infection and by interactions with the host’s immune system (236). Given the above, the parasite strain association is more important than the DTU, and the composition of the infecting parasite population plays an important role in the host response against T. cruzi as well as in the outcomes and severity of infection (89).
As final remarks, despite these findings, it is still difficult to establish a correlation between the genetic diversity of T. cruzi and the clinical manifestations of Chagas disease. Although there was some experimental support for the differential tissue tropism of T. cruzi strains (126), other reports failed to link parasite genetics to the clinical forms of Chagas disease (41, 220, 237). The genetic aspects of parasites may play an essential role in determining which host tissues will be infected and thus influence the pathogenesis of the disease. However, it is important to carry out studies that evaluate the behavior of different isolates in co-infections (115), considering that this interaction could modify the intrinsic behavior of individual isolates, changing the origin of the infection in Chagas disease. Developing tools capable of accessing intra-group genetic and gene expression variability is also necessary.
6 Immunosuppression and reactivation of the Chagas disease
Reactivation in Chagas disease occurs when T. cruzi escapes from the host’s immune system, resulting in multiplication, tissue inflammation, and increased blood parasitism in chronic infections (55, 238). This process is probably due to an imbalance in the relationship between the parasite and host when the cellular immune system is affected, where parasite persistence has been associated with myocarditis progression (61). In most cases, immunosuppression modifies pre-existing diseases, leading to developing or reactivating opportunistic infections, which present special and often severe clinical features (239).
Cases of reactivation of infectious parasitic diseases have been commonly recorded, such as Chagas disease, visceral leishmaniasis, and toxoplasmosis, in transplant patients treated with immunosuppressives in organ transplants or blood transfusions (240, 241) and in cases of co-infection with human immunodeficiency virus (HIV) (54, 242).
Since the 1990s, there have been reports of reactivation of chronic Chagas disease in individuals infected with HIV (238, 243–245). In these patients, T. cruzi most commonly affects the central nervous system (75%–80% of cases) and is often associated with cardiomyopathy (246, 247). The clinical manifestations included acute meningoencephalitis, fever, focal neurological deficits, and histopathological examination revealing inflammation with amastigotes in glial cells and neurons (248). Individuals may also present with cardiac symptoms (25% to 44% of cases), such as heart failure and arrhythmias.
Despite the fact that Chagas disease is a lifelong infection, the anti-trypanosomal therapy for infected people during the chronic phase of the disease is not clearly effective and remains a challenge (249). Heart transplantation is a therapeutic option for those patients with advanced heart failure refractory to medical therapy. Reactivation of Chagas disease is a common finding under immunosuppressive conditions, such as AIDS, autoimmune diseases, cancer (and the chemotherapy used to treat it), and, obviously, pharmacological immunosuppression to avoid allograph rejection (250–252).
Severity of the acute phase of T. cruzi infection is closely related to aggressive immunosuppression. Type I T helper immune response is an important mechanism involved in the T. cruzi infection (185). It is well established that a high immunossupression is able to modify the cytokine profile of type I T helper lymphocytes to Th2-type response. This environment constitutes a favorable condition for exacerbation of the disease and its symptoms including fever, anorexia, myalgia, diarrhea, panniculitis, myocarditis, meningoencephalitis, and encephalitis (252). In this context, some T. cruzi DTUs seem to contribute to increase the risk of certain morbidities in comparison to others. On the other hand, since host–pathogen interactions are complex, with several unknown aspects influencing the fate of infections, the interplay between the pathogen and the host’s immune response must be critical in determining disease evolution (252). The alignment between the distribution of T. cruzi DTUs and disease severity has led to a general association of TcI with oral transmission and severe acute cases in the North and Central Americas and the Amazon region in Brazil; TcIII and TcIV associated with oral transmission and acute cases in the Amazon region in Brazil and Venezuela, respectively (252).
Considering the natural history of Chagas disease, approximately 30% of T. cruzi-infected individuals develop progressive destruction of the myocardium, which causes chronic chagasic cardiomyopathy. The progress of the inflammatory process will debilitate the patient and enhance the chances of requiring a heart transplant, which becomes the only treatment option. However, reactivation of the disease may occur due to concomitant immunosuppressive therapy (249, 253, 254).
It has been reported to occur in 26.5% to 42.9% of patients who underwent cardiac transplantation (255, 256), ranging from asymptomatic to the presence of symptoms such as fever, subcutaneous involvement, and myocarditis (257). Sánchez-Valdéz et al. (2018) (258) documented the development of non-proliferating intracellular amastigotes of T. cruzi (dormancy state), which were uniquely resistant to extended drug treatment and could re-establish infection after as many as 30 days of drug exposure. This finding may help understand the failure of highly cytotoxic compounds to completely resolve the infection in chronic patients and make the relationship between immunosuppression and Chagas disease worrying.
Several teams have investigated the correlation between T. cruzi DTU and Chagas disease reactivation (28, 54, 259–262). Burgos et al. (2008) (263) identified mixed infection by T. cruzi I and T. cruzi IV/V in blood, but only T. cruzi I was found at the site of CNS reactivation. Costales et al. (2015) (264) and Inga and Oliveira (2019) (265) related the reactivation of the disease to the parasites of the TcI, as previously found in cases of reactivation by HIV co-infection (263). Cura et al. (2012) (266) demonstrated that the distribution of parasites in tissues and blood differs between immunocompetent patients and cardiac transplant patients with reactivation. This result may reflect the unmasking of mixed infections below the detection level before reactivation. The authors showed that TcI was more frequent in the bloodstream and cardiac tissues from immunosuppressed patients, while TcI and TcII/VI were detected in skin biopsy slices in a kidney transplant patient. Recently, Marcon et al. (2022) (262) investigated the DTU present in the blood of T. cruzi/HIV co-infected patients with antiretroviral infection in Brazil and showed that the majority of them were infected with TcII and one case had mixed infection (TcII and TcV/TcVI).
Experimental models have shown that the severity of pathogenesis and tissue parasitism during chronic reactivated disease resembles the symptoms presented in the acute phase of infection. Immunodeficient mice infected with T. cruzi showed higher parasitemia and myocardial parasitism with inflammation than mice infected with T. cruzi without immunosuppression (267). Santos et al. (2010) (86) observed a distinct pattern, demonstrating that the T. cruzi genetic lineage, in combination with specific treatment with benznidazole, plays an important role in the reactivation of infection in immunosuppressed mice. The authors observed that animals infected with TcII showed reactivation in only 5% of cases. In comparison, those infected with TcI clones showed reactivation in greater than 51% of the animals when immunosuppressed with cyclophosphamide. These data reinforce the strong presence of TcI DTU in cases of Chagas disease in clinical studies.
Nonetheless, in reactivated Chagas disease, several T. cruzi DTUs have been detected by molecular methods, as previously discussed. It is currently impossible to establish a strict correlation between specific DTU and Chagas disease immunosuppression (28, 54, 61, 259–261). The DTU identified seems to reflect the more common genotype in a defined geographic region. Sales-Campos et al. (2014) (127) observed that the behavior of strains with the same DTU can be different in immunosuppressed mice. These data reinforce the idea that the composition of the infecting parasite population plays an essential role in host–parasite interactions (89, 96, 268).
The biological and genetic heterogeneity of circulating strains of T. cruzi can make it challenging to interpret the different manifestations of the disease and determine the correlation between DTU and specific clinical traits. Burgos et al. (2010) (61) showed distinct T. cruzi signatures in cardiac explant specimens and blood samples obtained from a cohort of 16 Argentinean patients with cardiac disease who underwent transplantation. TcI DTU was observed in three explant samples, and TcII, TcV, and TcVI were observed in five samples. Post-heart transplantation follow-up identified TcI in five patients and TcII, TcV, and TcVI in seven patients. TcI, TcV, and TcVI were detected in skin chorioma specimens, and TcV and TcVI were detected in samples obtained from patients with myocarditis reactivation.
However, it should be considered that this is a complex process involving several host and parasite factors. It is reasonable to suppose that the immunological condition of the patient, whether associated or not with the reactivation of the infection, and the strain of the parasite may have an important role during the course of the disease.
Considering the data presented, it is reasonable to suppose that the immunological condition of the patient, whether associated or not with the infection’s reactivation and the parasite’s strain, may have an important role during the Chagas disease reactivation process (261). Thus, considering the large number of infected patients, the increasing cases of immunosuppression, and the severity of the disease in this situation, we emphasize that the role of genetic and biological aspects of T. cruzi on Chagas disease reactivation needs to be further explored in basic and clinical research.
7 Influence of T. cruzi DTUs in congenital transmission of the Chagas disease
Congenital transmission has emerged as a significant pathway for the dissemination of T. cruzi, presenting a global challenge that endures even in nations equipped with robust vector control programs and established blood banks (269, 270), and represents the predominant mode of transmission in non-endemic regions (271–274).
A comprehensive meta-analysis by Howard et al. (2014) (275) assessed the frequency of congenital transmission, revealing a 5% infection rate among chagasic mothers. The risk of vertical transmission exhibits considerable disparity, ranging from 0.75% to 28.6% across diverse geographic regions (275). The robustness and efficacy of congenital infection screening services significantly influence this aspect (275). Additionally, it has been proposed that genetic T. cruzi diversity is a pivotal factor in shaping this process (276). In support of this hypothesis, Cencig et al. (2013) (277) explored the impact of infection with three T. cruzi DTUs (TcI, TcII, and TcVI) on fertility, pregnancy, and congenital transmission. The authors observed that the parasite induced significant damage in the acute phase, irrespective of genotype, whereas in the chronic phase, the primary symptom was delayed uterine growth. Vertical transmission was detected in the acute phase of the infection, rare in the chronic phase, and associated with TcII and TcVI. Nevertheless, Alkmim-Oliveira et al. (2013) (278) experimentally assessed the potential for congenital transmission of TcI and TcV in female BALB/c mice. They observed that both genotypes could be transmitted to the fetus at rates of 58.1% and 46.6%, respectively. Females infected with TcI exhibited a lower average number of pups per female (4.8) than those infected with TcV (8.3).
A distinct association between transplacental transmission and specific T. cruzi DTU is not evident in human infections. These data substantiate the hypothesis that the results reflect the most prevalent DTU within these regions, given that most DTUs have been discerned in human cases of congenital infection. For example, in Bolivia and Argentina, TcV predominantly constituted the detected DTU, although TcII and TcVI have also been documented (278–282). These genotypes are recurrently linked to vertical transmission across the Southern Cone of America (278, 283–286). TcI has been documented in Mexico, Central American countries, and Colombia (287–289) and has been detected in congenital infection cases in Argentina and Chile (285, 286). Herrera et al. (2019) (289) revealed a higher frequency of TcII-TcV-TcVI than TcI in Honduras and Mexico, implying that the prevalence of these genotypes in these regions may be more extensive than previously acknowledged.
Moreover, several studies have identified that mixed T. cruzi infections involving different DTUs or haplotypes within the same DTU are frequently detected (281, 282, 289, 290). A significant aspect of mixed infections is the detection of genetic profiles in infants that differ from those observed in the mother (282, 290). This suggests that the occurrence of polyclonal strains is common, highlighting that the dynamics of the T. cruzi population are profoundly influenced by both the host and the environment.
In Brazil, genotyping of T. cruzi from congenital infections is limited, likely reflecting the country’s deficient structure of congenital infection surveillance and patient management (291). Bittencourt et al. (1985) (292) characterized isoenzymes of parasites isolated from mothers and infants from Bahia state. Their observations revealed that, except for one triple heterozygous GPI pattern, all the identified patterns were associated with zymodeme 2. This suggests the probable occurrence of TcII, representing the primary genotype infecting patients in the central and southern regions or, secondarily, TcVI. Data from our research team on Chagas disease in the Jequitinhonha Valley, Brazil, MG, coordinated by Marta de Lana, PhD, support this information through the genotyping of strains obtained from mothers and children in the northwest region of Minas Gerais. The results revealed identical molecular profiles between the paired strains, all isolates were identified as TcII, and similar ones were found most frequently in this region (67). Llewellyn et al. (2015) (290) also detected TcII in cases of congenital infection in the Goias state. TcIV was first identified in association with a potential case of congenital infection in the northern region of Brazil, specifically in the state of Pará (293). This was linked to a maternal infection during the oral transmission outbreak. The authors also documented a case associated with TcI, and the findings once again reflected the DTU most frequently observed in transmission cycles within the region (39). TcIII and TcIV were less often associated with congenital infections, likely due to their lower occurrence in T. cruzi domestic transmission cycles.
The studies mentioned above showed that T. cruzi genetic diversity alone is inadequate to elucidate the incidence of congenital infection, even more so considering the intense molecular and biological polymorphisms observed in the species and that other factors play a crucial role in the success of this infection. The probability of vertical transmission by T. cruzi is affected by the infection stage, reinfection, maternal immune response, placental factors, and parasite characteristics such as infectivity, virulence, and tissue tropism (294–298).
Within the framework of T. cruzi subpopulations, certain studies have revealed that different strains exhibit distinct capabilities to infect placental tissues. For instance, Andrade (1982) (299) emphasized the significant influence of parasite strain on congenital T. cruzi infection. The presence of parasites in the placenta was infrequent and limited in animals infected with Type I and II strains (Y, Peruvian, and Honorina). In contrast, it was intense and frequent in those infected with the Colombian strain (Type III). The authors also noted that the probability of placental infection appears more plausible during peak parasitemia in the acute phase than in the chronic phase. Medina et al. (2018) (300) demonstrated notable variations in the infectivity and pathogenicity of two T. cruzi strains within the placental cells of mice. This underscores the significance of the intrinsic characteristics of T. cruzi stocks and their interactions with the host in determining the success of congenital infections. These findings indicated that the VD strain (TcVI) exhibited higher infectivity and pathogenicity than the Y strain (TcII).
However, few studies have assessed the behavior of DTUs in experimental congenital infections that have been conducted, often confined to one or two T. cruzi genotypes. To comprehensively understand the influence of the intrinsic characteristics of the parasite on congenital transmission, it is necessary to investigate a more extensive array of strains from all DTUs, encompassing their diverse biological properties. Furthermore, it should be noted that some studies have shown important genetic variability within the same DTU associated with congenital transmission (283, 289, 290). Burgos et al. (2009) (283) showed different minicircle signatures in T. cruzi stocks belonging to the same lineages in Chaco Province, Argentina. Herrera et al. (2019) (289) confirmed that analyzing intra-DTU genetic variability may provide more informative insights into its correlation with congenital infection. In their study on T. cruzi stocks isolated from the umbilical cord blood of newborns, the authors observed the presence of TcI, TcII, TcV, and mixed infections circulating in Argentina, Honduras, and Mexico. Through detailed haplotype sequencing of isolated parasites, they observed the circulation of identical haplotypes in these countries at varying rates. For the first time, a strong association was observed between parasite haplotypes and congenital infection, suggesting that identifying parasite haplotypes in pregnant women could predict the vertical transmission of T. cruzi in this geographic area.
Remarkably, the interaction between T. cruzi subpopulations and the host often elicits tissue and cellular responses that can significantly influence the course of congenital infection (295). Several studies have reported the modulation of gene expression in the placenta due to infection. Castilho et al. (2017, 2018) (301, 302) observed alterations in the expression of genes associated with innate immune response during ex vivo infection of human placental explants. Initially, they investigated the response of human placental chorionic villi explants to infection with T. cruzi (Y strain) and Trypanosoma gondii. They found that T. cruzi infection is linked to the expression and activation of TLR-2, while T. gondii infection is mediated by TLR-4 and TLR-9. Inhibition of these receptors increases the DNA parasite load in placental explants.
Additionally, T. cruzi induces increases in the expression and secretion of the cytokines TNF-α, IL-1β, IL-6, IL-8, and IL-10, whereas T. gondii increases the expression of TNF-α and CXCL8 but only elevates the secretion of IL-8 (301). Additional studies have revealed that genes involved in complement regulation and function, such as CD46 and C1q, were upregulated in T. cruzi infection. Furthermore, TLR7 and TLR8 showed increased expression, along with the cytokine IL-6, dependent on the parasite burden (302). The immune response profile elicited by the parasite likely affects its capacity to establish and regulate damage (303). Therefore, the local response of the placenta to infection is intricately linked to the risk of congenital transmission and can be influenced by factors such as the host’s strain, parasite load, and genetic background (296–298, 304). Juiz et al. (2017) (305) also demonstrated that T. cruzi infection regulates genes associated with innate immunity and the response to interferon-gamma when studying VD and K38 strains in C57Bl/6J mice. The authors observed that this regulatory process was strain-dependent. In infections by the VD strain, increased placental tropism and a robust immune response were detected, potentially reducing the risk of congenital transmission at the expense of cellular metabolism. Maternal parasitemia is another crucial factor associated with congenital risk of transmission.
These findings underscore the notion that congenital transmission of T. cruzi is a complex and orchestrated process, wherein various characteristics of both the parasite and the host play pivotal roles in the success of placental infection and subsequent transmission to the fetus. On the other hand, the immune response mechanisms revealed during infection safeguard the placenta from parasitic infiltration, thereby reducing parasitic load and minimizing the risk of congenital infection. Congenital infection has been linked to premature birth, low birth weight, developmental delay, and elevated mortality rates. Moreover, 30% of the infants infected at birth may manifest symptomatic forms in the future. Given the extensive geographical distribution of the parasite and the substantial number of mothers affected by T. cruzi, establishing a robust healthcare network is essential. This network should facilitate serological screening of pregnant women, ensuring prompt diagnosis and treatment of newborns. Furthermore, studies that explore the risk markers and prognoses of congenital infections that span diverse geographic regions are necessary. Vertical transmission is not explicitly associated with a particular T. cruzi DTU, manifesting through various genetic groups in distinct geographic regions. Most investigations are concentrated in Argentina and Bolivia, mainly focusing on TcV. Expanding the surveillance of congenital transmission and broadening the examination of strains/genetic groups prevalent in other areas is essential to better understand transmission risks and develop intervention proposals.
8 Impact of T. cruzi DTUs in the Chagas disease treatment
Since the beginning of the 1970s, two drugs have been used for the treatment of T. cruzi infection: nifurtimox (NF) (launched by Bayer in 1967, Lampit®) and benznidazole (BZ) (launched by Roche in 1972, Rochagan® and Radanil®) (306). These nitroheterocyclic compounds require prolonged treatment in patients infected with T. cruzi (frequently 60 days) and usually exhibit undesirable adverse reactions that hamper treatment continuity (306, 307). The therapeutic effectiveness of these two drugs varies according to the phase of infection in the patients with Chagas disease (308–310). Numerous studies have reported up to 80% parasitological cure in the acute phase (311) and 60%–70% cure in the recent chronic phase of the disease, in children up to 14 years of age (312–315). However, the major limitation of Chagas disease treatment is its low efficacy in the chronic phase, with only 5%–20% of patients considered cured, as assessed by ≥20 years or more follow-up (306, 310, 316–320). However, benznidazole treatment in the chronic phase can improve the prognosis and clinical outcome in patients with Chagas cardiomyopathy (314, 321–323).
The high variability in the efficacy of benznidazole and NF treatments is controversial, and some studies have attributed it to T. cruzi genetic factors. This could explain the differences observed in the screening results when comparing distinct geographic areas (112, 268, 322). The clonal evolution model postulated for T. cruzi (266) indicated an association between the phylogenetic divergence of parasite clones and the natural variation in drug susceptibility among T. cruzi strains, which is considered an important factor that explains the therapeutic failure in some treated patients with Chagas disease (85, 324–328). Brener et al. (1976) (329) were the first to indicate the heterogeneity of the responses of T. cruzi strains to specific treatments. A pioneering study (325) demonstrated a large gradient of drug efficacy for nifurtimox and benznidazole (from 0% to 100%) when 47 T. cruzi strains isolated from humans, vectors, and sylvatic reservoirs were investigated in murine models. According to this study, Andrade (1985) (112) observed that during the acute phase, hybrid T. cruzi strains were highly susceptible, T. cruzi II (TcII) strains were partially to highly susceptible, and T. cruzi I (TcI) strains were highly resistant to benznidazole and nifurtimox. Corroborating this study, the authors also verified greater parasitological test negativity in mice infected with Type III (TcI) strains (85.3%) compared to mice infected with Type II (TcII) strains (43%) in the chronic phase after treatment with benznidazole. In animals in the chronic phase, treated with nifurtimox and infected with Type III (TcI) and Type II (TcII) strains, parasitological test negativity was 71.4% and 66%, respectively (330). Similarly, Andrade et al. (1992) (326) demonstrated that in mice treated with benznidazole or benznidazole plus nifurtimox, the cure rate was 66%–100% in those infected with Type II strains (TcII), while in the animals infected with Type III strains (TcI), the cure rate was 0%–9%. The authors indicated that the correlation between the treatment results in patients and mice was 81.8% (9 of 11 cases). Similarly, Filardi and Brener (1987) (324) reported natural resistance to drugs used to treat T. cruzi infection in strains or clones isolated from both wild and domestic cycles. T. cruzi strains from different geographical areas and isolated from patients with chronic Chagas disease, triatomines, and wild reservoirs were inoculated into mice and showed a gradient of drug susceptibility ranging from 0% to 100%. The correlation between T. cruzi strains and the treatment response of Chagas disease was evaluated by Guedes et al. (2002) (331) using dogs as experimental models. The researchers demonstrated that all dogs infected with T. cruzi Y strain (TcII) and treated with benznidazole in the acute phase were cured. Moreover, five animals showed antibody levels similar to those of untreated controls, one infected with Berenice-78 (TcII), and four with Colombian strain (TcI), while complement-mediated lysis (CoML) was persistently positive 6 months post-treatment in five dogs, one of which was infected with Berenice-78 (TcII) and four with the Colombian strain (TcI). This study showed that T. cruzi genetic variability probably influenced the treatment response, as T. cruzi strains from TcI DTU showed more significant resistance to treatment with benznidazole and nifurtimox than T. cruzi strains from TcII DTU.
Some authors also consider that T. cruzi genetic aspects can explain the differences observed after treatment in distinct geographic areas (7, 315, 332). Yun et al. (2009) (315) showed that in patients with Chagas disease from Honduras, Guatemala, and Bolivia treated with benznidazole, the seroconversion varied widely, even within the same country. Different parasite lineages in distinct geographic regions may explain the differences in seroconversion rates.
Toledo et al. (2003) (85), in a well-designed research, evaluated 20 T. cruzi clones isolated from vectors and hosts from different endemic regions and tested the benznidazole and itraconazole susceptibilities during the acute and chronic phases of experimental infection in BALB/c mice. The authors found differences in susceptibility to treatment between the T. cruzi stocks according to the genotype, phase at infection, and the drug used in this work. Mice infected with genotype 19 (TcI) or genotype 39 (hybrid) were partially susceptible, while those infected with genotype 20 (TcI) were resistant, and those infected with genotype 32 (TcII) were susceptible to treatment with benznidazole in both infection phases. Considering the treatment with itraconazole drug, mice infected with genotype 19 (TcI), 20 (TcI), or 39 (hybrid) were resistant in the acute phase, and those infected with genotype 32 (TcII) were partially susceptible to treatment. In the chronic phase, animals infected with genotype 19 (TcI) or genotype 20 (TcI) were resistant, those infected with genotype 39 (hybrid) were partially susceptible, and those infected with genotype 32 (TcII) were susceptible to itraconazole treatment. In general, this study demonstrated that clones that belong to TcI DTU were resistant to benznidazole, mainly genotype 20 (TcI), whereas the resistance to itraconazole was observed in both genotypes 20 (TcI) and 19 (TcI). Moreover, genotype 32 (TcII) showed a typical susceptibility profile to benznidazole and itraconazole. Furthermore, Toledo et al. (2004) (333) observed that specific treatment of T. cruzi infection altered the parasitological and histopathological parameters according to the parasite genotype (333). Animals infected with genotype 19 (TcI) showed a reduction in tissue parasitism, whereas those infected with genotypes 20 (TcI) and 32 (TcII) showed a decrease in the inflammatory process after benznidazole treatment in the acute phase. In this study, mice infected with genotype 39 (TcV) showed reduced tissue parasitism after treatment with benznidazole in the chronic phase. These data corroborate previous results that indicated that TcI DTU is more resistant to treatment, but in contrast also revealed a positive impact of benznidazole treatment in the pathogenesis of mice infected with this genetic group, even though there is no parasitological cure. The biological parameters of T. cruzi clones belonging to different genotypes were also tested in the treatment response to T. cruzi infection in vitro (99, 334). Revollo et al. (1998) (99) investigated the susceptibility to treatment of T. cruzi stocks isolated from various ecological cycles, places, and hosts. They showed that genetic groups 19 (TcI) and 20 (TcI) of the epimastigote and amastigote forms of T. cruzi were less sensitive to treatment with benznidazole or nifurtimox. Nonetheless, some studies have encountered challenges establishing a definitive correlation between T. cruzi DTU and therapeutic response in Chagas disease. These findings imply that additional factors play an important role in the observed variations in therapeutic efficacy among patients with Chagas disease (335–337). Murta et al. (1998) (335) observed that only strains classified as zymodeme ZB (hybrid) exhibited susceptibility to benznidazole and nifurtimox treatment, whereas zymodemes Z1 (TcI) or Z2 (TcII) displayed a diverse pattern in treatment response, being either susceptible or naturally resistant to these nitroderivatives. Similarly, Moreno et al. (2010) (337) did not find a correlation between in vitro drug susceptibility and T. cruzi. The authors conducted in vitro evaluations of benznidazole sensitivity in T. cruzi isolates obtained from seven chronic patients before benznidazole administration and at various intervals after therapy. All isolates were typed as TcII, and three patients (3/7) were considered cured.
Villarreal et al. (2004) (336) investigated potential correlations between in vitro benznidazole susceptibility and T. cruzi genetic diversity. This study demonstrated that the natural susceptibility of T. cruzi, expressed as IC50 (concentration achieving a 50% inhibition of parasite growth), was not associated with its genetic structure represented by distinct DTUs. The authors concluded that this result likely stems from the high intra-DTU variation of the surveyed IC50 values.
In the context of Chagas disease, the clinical and epidemiological landscapes in the Brazilian Amazon markedly differ from classical endemic transmission areas, possibly influenced by the genetic and biological characteristics of circulating T. cruzi DTUs in this region (117). Multiple studies have investigated the susceptibility of T. cruzi strains from the Amazon to benznidazole (117, 118, 338). Monteiro et al. (2013) (117) examined T. cruzi isolates from the Western Amazon Region that were attributed to TcI and TcIV in Swiss mice. They observed that, although TcI exhibited higher mortality rates in the early chronic phase, a greater frequency of mice with inflammatory processes in any organ, and a higher frequency of mice with tissue parasitism, this DTU showed more susceptibility to benznidazole than TcIV. Teston et al. (2013) (118) investigated the susceptibility of natural T. cruzi populations from the state of Amazonas, belonging to TcI and TcIV, in comparison with strains of TcI and TcII DTUs from the states of Paraná and Minas Gerais. Although the researchers found no clear association between susceptibility to benznidazole and T. cruzi DTUs, it is demonstrated that TcIV strains exhibited more significant reductions in parasitological, molecular, and serological parameters after treatment than TcI and TcII strains. Notably, TcI strains from Amazonas were significantly more sensitive to benznidazole than TcI strains from Paraná. The extensive genetic variability of TcI isolates from different geographical regions supports the proposition that at least four haplotypes are associated with distinct transmission cycles of the parasite (123, 287, 339, 340). Studies assessing the response to specific chemotherapy in patients from outbreaks related to oral transmission in areas predominantly inhabited by TcI in the Amazon Region demonstrated high parasitological cure rates and decreased antibody titers (341, 342). This evidence supports the notion that TcI isolates from the Brazilian Amazon exhibit dissimilar behavior compared to other regions. The genetic heterogeneity of the TcI suggests that correlations between genetic and biological properties should be investigated at the sub-DTU level. Consistent with the findings of Teston et al. (2013) (118), Gruendling et al. (2015) (338) found no variation in parasitemia, infectivity, or mortality among DTUs when comparing the impact of benznidazole treatment during the acute phase in mice inoculated with TcI and TcIV strains from Amazonas and TcII strains from Paraná, but the proportion of parasitized organs varied with the genetic group.
Disparities in the therapeutic response to Chagas disease treatment have been demonstrated within geographical regions that predominantly harbor the same T. cruzi DTU (100, 343). Oliveira-Silva et al. (2015) (343) evaluated the efficacy of benznidazole in both acute and chronic phases in mice infected with TcII isolated from children in the Jequitinhonha Valley, state of Minas Gerais, Brazil, before treatment initiation. This study revealed that most strains resisted benznidazole in both phases, as evidenced by positive parasitological and/or serological tests. Nevertheless, the authors concluded that the treatment yielded benefits, noting a reduction and/or suppression of parasitemia in mice infected with all strains during the acute phase, associated with a reduction/elimination of inflammation and fibrosis. These findings contrast with those of Toledo et al. (2003) (85), who studied clonal stocks of T. cruzi with different genotypes, including TcII (equivalent to genotype 32), and reported cure rates of 80% and 69.2% in mice treated during the acute and chronic phases, respectively. Variance in the drug response of T. cruzi TcII strains could be attributed to the distinct geographical origins of the parasite populations used in the two studies (343). In another study, Oliveira et al. (2017) (100) assessed the susceptibility of mice to nifurtimox treatment using strains isolated from patients with chronic Chagas disease from the same region of Berilo in the Jequitinhonha Valley. Consistent with the observations of Oliveira-Silva et al. (2015) (343), they noted that only 25% of mice infected with the TcII strain and treated during the acute phase presented negative enzyme-linked immunosorbent assay (ELISA) results in 180 days post-treatment (100). However, the researchers concluded that the treatment had a positive impact, as animals infected with TcII or TcVI exhibited a reduction or elimination of parasitemia and inflammatory lesions.
Various drugs have been tested as alternatives to nitroderivatives against a range of T. cruzi strains (344, 345). Molina et al. (2000) (344) investigated the in vivo activity of posaconazole against a series of T. cruzi strains in both immunocompetent and immunosuppressed murine hosts. The results of this study revealed that in mice infected with the CL strain (TcVI), posaconazole at 20 mg/kg/day successfully cured 100% of the surviving animals, with a cure rate comparable to that achieved with benznidazole at 100 mg/kg/day. In contrast, mice infected with the Y strain (TcII) exhibited a cure rate of less than 50% with 100 mg/kg/day benznidazole, whereas the cure rate for animals receiving 20 mg/kg/day posaconazole approached 90%. Notably, in mice infected with the Colombian strain (TcI), the benznidazole at 100 mg/kg/day failed to induce a parasitological cure. However, 50% of the surviving animals that received posaconazole at a dose of 20 mg/kg/day were successfully cured. The authors suggested that posaconazole was effective against both susceptible and drug-resistant parasite populations in murine hosts. To ascertain the effectiveness of current and prospective drugs against diverse T. cruzi stocks, Moraes et al. (2014) (345) evaluated the in vitro activities of different compounds against six T. cruzi DTUs. Major differences in the susceptibility or resistance of strains and clones to the tested drugs were not found, indicating that DTUs may not directly correlate with a specific medication resistance pattern in vitro.
Additionally, ergosterol biosynthesis inhibitors have been introduced as new chemotherapeutic agents for treating Chagas disease. Triazoles such as posaconazole and ravuconazole, essential for ergosterol biosynthesis, have demonstrated potent trypanocidal activity both in vitro and in vivo (201, 344, 346–350). Among the most advanced and promising compounds is another nitroheterocyclic compound, fexinidazole, which exhibits activity against T. cruzi (351). Parallel efforts led to the discovery of the oxaborole class, specifically AN4169 (SCYX-6759), which demonstrated curative antichagasic activity in a mouse model and is now positioned as an innovative drug for the treatment of Chagas disease (352).
Collectively, these studies demonstrate that the genetic variability of T. cruzi alone is insufficient to account for the variations observed in treatment responses comprehensively. An essential consideration in Chagas disease is the occurrence of mixed infections in vertebrate and invertebrate hosts (353, 354). Substantial interactions within the subpopulations of these mixtures have been observed, leading to alterations in the biological properties of the parasite (94, 104, 105, 355). Such changes can potentially influence morbidity, the dynamics of T. cruzi transmission, and the response to chemotherapy in Chagas disease. Martins et al. (2007) (95) investigated the consequences of dual infections with clonal stocks of T. cruzi genotypes on the efficacy of benznidazole treatment in a murine model. Their investigation revealed that mixed infections exhibited treatment responses distinct from single-infection analyses’ predictions. The authors verified that 9 out of 24 dual infections shifted the predicted benznidazole susceptibility profile compared to the respective single infections. Notably, six dual infections involving almost all tested genotype combinations demonstrated an increase in the cure rate, except for 19 + 32 (TcI + TcII) and 20 + 19 (TcI + TcI), while two exhibited a reduction, including genotypes 19 + 39 (TcI + TcV) and 19 + 32 (TcI + TcII). The researchers concluded that mixed T. cruzi infections, a phenomenon observed in nature, could significantly impact the efficacy of chemotherapy for Chagas disease.
Some studies suggest that additional factors should be considered when assessing the response to Chagas disease treatment (99, 334). Different life stages of the parasite can influence the susceptibility of various T. cruzi strains to benznidazole and nifurtimox (334, 356, 357). Canavaci et al. (2010) (356) demonstrated that the in vitro susceptibility of intracellular amastigotes of CL and Colombian strains is indistinguishable even though these strains are well known for their susceptibility and high resistance to benznidazole and nifurtimox in vivo, respectively (324, 344). Revollo et al. (2019) (334) explored the in vitro susceptibility of 21 T. cruzi strains belonging to the TcI, TcII, and TcV DTUs to epimastigotes, trypomastigotes, and amastigotes. These strains were isolated from patients, reservoirs, and triatomine bugs with distinct geographic origins. The study employed a methodology based on computing the epidemiological cutoff value (COwt) of T. cruzi stages against benznidazole and nifurtimox. Using a panel of previously characterized strains, the researchers examined the frequency of the sensitive (wild-type) phenotype within the three DTUs. The findings revealed that epimastigotes are more susceptible to both drugs than intracellular amastigotes, whereas trypomastigotes globally exhibit a higher inherent capacity to resist the effects of both benznidazole and nifurtimox. Moreover, regardless of the stage studied, strains belonging to TcI were more frequently identified as less susceptible to treatment than TcII and TcV. The drug susceptibility of T. cruzi is related to the genotype but is also linked to the life history trait of each strain (334).
Recently, a stage in the intracellular life cycle of T. cruzi, referred to as dormant amastigotes (258, 358), has been identified, which appears to influence the response to treatment. In addition to variations in their replicative capacity, these dormant amastigotes exhibit differences in their transcriptomes and more significant resistance to treatment during their non-replicative stages (358, 359). The authors demonstrated that this phenotype is transient, and dormant amastigotes revert to actively replicating forms susceptible to the drug. Consequently, trypanocidal drugs efficiently eliminate actively replicating amastigotes but not dormant forms, suggesting that dormancy is the primary factor contributing to the ineffectiveness of these drugs in achieving a consistent parasitological cure (360). Therefore, a treatment protocol combining high doses, reduced frequency, and extended treatment duration would likely be more effective in addressing and eliminating dormant parasites (360) and achieving therapeutic success.
Indeed, the response to treatment for Chagas disease is understood to be a multifactorial and intricate process with numerous gaps yet to be addressed. Therapeutic failure encompasses a set of factors associated with the host (including genetic and immunological traits), infective agent (genetics and acquired drug resistance), drug used (pharmacodynamics/pharmacokinetics), and chemotherapeutic protocol (334). Differences in assay methodology, including the life cycle stage of the parasite used in the test and variation in T. cruzi strain population composition among different laboratories, should be duly considered (99, 334, 345). Additionally, it is crucial to compare the in vitro and in vivo assays. Given that most reports are based on mouse models, it is impossible to definitively attribute therapeutic failure to “natural” intrinsic T. cruzi resistance (345). In vivo chemotherapy involves several parameters that are often uncontrolled even in experimental models, such as the relationship between exposure and cure, route of administration, time of treatment, treatment regimen and length, mouse lineage, and type of immune response, along with other Chagas disease-related challenges, such as definitions of cure criteria and disease stage (345). It is also essential to consider the lesser phylogenetic subdivisions because of the heterogeneous behavior of strains within the same broad genetic subdivisions (85, 95). The biological characteristics of the parasite, such as susceptibility to treatment, may vary even within the same T. cruzi DTU (100, 343, 361). Because of the myriad factors that can impact the therapeutic response to Chagas disease, a priority in scientific research should be the development of new drugs with improved efficacy, shorter treatment course, fewer side effects, and resilience against T. cruzi genetic variability and dormant forms.
9 Methods used for the genotype-specific diagnosis of Chagas disease
The methodologies used for the genotype-specific diagnosis of Chagas disease to recognize T. cruzi DTUs have been developed, including biochemical and molecular methods (5, 7) as well as serological tests (362–371). Considering the distinct geographic distribution of DTUs (7), genotype-specific diagnosis of Chagas disease is relevant for implementing epidemiological surveillance and more precise strategies for disease control in endemic areas. Moreover, genotyping methods can also be helpful in establishing the clinical prognosis status of ongoing infection since different T. cruzi DTUs have been associated with distinct severity of Chagas disease (20, 72, 372). In addition, considering the divergent susceptibility of T. cruzi DTUs to the etiological treatment of Chagas disease (20, 85, 95), the methodologies used for the genotype-specific diagnosis of T. cruzi infection are important for post-therapeutic monitoring of treated patients.
T. cruzi genetic variability may interfere with the sensitivity of the methods used for Chagas disease diagnosis, mainly in serological tests. Previous studies have shown that patients from different geographic areas infected with distinct T. cruzi DTUs seem to display differential serological patterns upon diagnosis of Chagas disease (373–376). Verani et al. (2009) (375) verified two serological tests using serum samples from Bolívia and Peru, with distinct sensitivities ranging from 26.6% to 87.5%, corroborating the hypothesis that the intrinsic features of regional T. cruzi strains can impact serological tests. Thus, considering the genetic variability of T. cruzi, it is important that the antigens for the universal diagnosis of Chagas disease are expressed in different DTUs (376).
Currently, molecular methods are broadly used for genotype-specific diagnosis of single and mixed T. cruzi infections during the acute and chronic phases (5). Classification of T. cruzi in genetic groups was based on a variety of molecular targets by several techniques such as polymorphism of rDNA and mini-exon (377, 378), multilocus enzyme electrophoresis (MLEE) (4, 21, 379), multilocus sequence typing (MLST) (47, 380–382), restriction fragment length polymorphism (PCR-RFLP) (11, 276, 383, 384), random amplified polymorphic DNA (RAPD) (385), microsatellite typing (MLMT) (340, 386–388), target-specific PCR (22, 61, 287), PCR-DNA blotting with hybridization assays (389–392), and amplicon deep sequencing (290).
The method of Lewis et al. (2009) (6) has been commonly used for T. cruzi genotyping. This method is based, in principle, on the analysis of the divergent domain D7 of 24Sα subunit rDNA (LSU rDNA) (16) and two restriction fragment length polymorphisms (PCR-RFLP) of HSP60 (heat shock protein) and GPI (glucose 6-phosphate isomerase) genes. Combining these three methodologies enables the typing of most T. cruzi strains with good reproducibility (6). Another method broadly used for T. cruzi genotyping was proposed by D´Ávilla et al. (2009) (228), which is based on the PCR-RFLP of the subunit II of the mitochondrial enzyme cytochrome oxidase (COII) gene region and the amplification of the intergenic region of the spliced leader (SL-IR) of the miniexon gene (276), as well as the analyses of the divergent domain D7 of 24Sα subunit rDNA (LSU rDNA). This criterion also showed good reproducibility and identified mixed infections with different T. cruzi genotypes (228). De Oliveira et al. (2015) (67) identified the genetic profile of 63 T. cruzi samples isolated from patients with chronic Chagas disease in two municipalities of Jequitinhonha Valley, MG, Brazil, using protocols proposed by Lewis et al. (2009) (6) and D´Ávilla et al. (2009) (228). The authors demonstrated that the criteria of Lewis et al. (2009) (6) identified 89% of the samples as TcII, but it was not possible to define the genetic identity of seven isolates, and the criteria proposed by D´Ávilla et al. (2009) (228) corroborated the classification as TcII for the same samples and defined the classification of the other seven as TcVI. Presently, the methods used for typing T. cruzi DTUs are based on polymorphism of the mini-exon gene (Spliced Leader) and the 24Sα and 18S ribosomal RNA (22). Multilocus PCR-RFLP (MLP), which analyzes the genetic polymorphism of different T. cruzi loci (27), MLEE (379), and RAPD (21) employing different markers and steps have also been used for parasite genotyping mainly in single T. cruzi infections. Other assays include the analysis of complex electrophoretic patterns generated by restriction polymorphisms in PCR-amplified genomic DNA (384, 393, 394). These methods are able to discriminate T. cruzi DTUs, but they are work- and time-consuming, and the interpretation of the results may be misleading. Some molecular methods can be used for T. cruzi genotyping directly from biological samples, such as the unique low astringency specific PCR primer technique (LSSP-PCR) (125, 212) by analysis of the rDNA polymorphism (276, 395) and examination of the microsatellite polymorphism (263, 396), which are important for the best understanding of the pathogenesis of Chagas disease.
Real-time quantitative PCR (qPCR) is able to amplify satellite DNA (SatDNA) sequences from the T. cruzi genome (397–399). The qPCR detects variations in the SatDNA sequences of different T. cruzi genetic groups; therefore, this variability must be considered when estimating the parasitic load. This technique showed different sensitivities between the different DTUs of the parasite: TcI < TcII < TcV and TcVI (398). Cura et al. (2015) (400) used the real-time PCR with TaqMan probes (MTq-PCR) to identify T. cruzi mixed infections with parasite isolates from humans, triatomines, and wild reservoirs. The Multiplex Real-Time PCR assay using TaqMan probes was useful for determining the T. cruzi DTUs of cultures, vectors, and blood samples from patients in the acute phase (384, 394). Muñoz-San et al. (2017) (401) used the real-time PCR for genotyping of 53 blood samples from individuals with chronic Chagas disease, and revealed that the method was able to characterize 67.9% of samples, with high detection of TcII, followed by TcI. Muñoz-San et al. (2018) (402) also genotyped 86 samples of patients with chronic Chagas disease from Chile by real-time PCR assay and determined whether T. cruzi DTUs are related to the presence of heart disease. The data obtained in this study showed that in the group with cardiomyopathy, the most frequent DTU was TcI (56.1%), followed by TcII (19.5%), while mixed infections of TcI and TcII were observed in 7.3% of the patients. In this study, in the group without cardiac pathologies, TcI and TcII were found at similar rates (28.9% and 31.1%, respectively), and mixed infections TcI + TcII were found in 17.8% of the cases. Ramírez et al. (2017) (403) analyzed 335 distinct T. cruzi SatDNA sequences from 19 T. cruzi stocks representative of TcI-TcVI DTUs by real-time PCR for phylogenetic inference. The authors revealed that all sequences were grouped into three major clusters, which corresponded to sequences from TcI/III, TcII, and TcIV, whereas the TcV and TcVI stocks had two sets of sequences distributed into TcI/III and TcII clusters. The major limitation of this approach used in this work is that it cannot distinguish between the presence of hybrid lineages TcV and TcVI and T. cruzi mixed infections with TcI or TcIII and TcII strains. Moreover, this study opens the possibility of typing samples from patients with Chagas disease with low parasitic loads and improves molecular diagnostic methods of T. cruzi infection based on SatDNA sequence amplification. This genotyping strategy could be useful for patients with chronic Chagas disease with low parasitic loads, whose samples are usually difficult to genotype (400, 404).
Increasingly, DNA markers to T. cruzi genotype with high copy numbers, such as kinetoplast minicircles, are needed because of the low number of parasites in biological samples, particularly in patients with chronic Chagas disease. Bontempi et al. (2016) (405) developed a DNA assay called minicircle lineage specific-PCR (MLS-PCR) to genotype reference strains of T. cruzi and parasite DTUs isolated directly from biological samples. The researchers verified the high specificity and sensitivity of the new approach for TcI, TcII, TcV, and TcVI genotyping in 22 T. cruzi reference strains. Moreover, the MLS-PCR technique and hybridization tests using specific kDNA probes could genotype 94% of the blood samples from patients with chronic Chagas disease in northeastern Argentina. Since there is frequently a low number of parasites in infected tissues or blood samples, genetic markers with a high number of copies are necessary to achieve good sensitivity of detection (406). Rusman et al. (2019) (407) performed deep amplicon sequencing of the hypervariable kDNA minicircles’ hypervariable region in nine strains of six T. cruzi DTUs. This work demonstrated that the number and diversity of mHVR clusters were variable among DTUs, and even within a DTU, T. cruzi strains of the same DTU shared more mHVR clusters than strains of different DTUs. The authors concluded that mHVR amplicon sequencing is a reproducible technique that allows multiplexed analysis of hundreds of strains by direct genotyping on biological samples, but has the disadvantage of being technically cumbersome, relying on visual interpretation of bands, and requiring representative strains of the diversity of T. cruzi in every assay (used as probes). Da Cruz Moreira and Ramirez (2019) (408) amplified several targets from the T. cruzi genome, such as SL-IRac, SL-IR I-II, 24Sα rDNA, and A10, by multilocus conventional PCR to genotype the DTUs directly from the blood and other clinical samples, without the need to isolate the parasite before DNA extraction, even at a lower parasite concentration.
One of the main challenges in genotyping biological samples is the identification of mixed infections by different T. cruzi DTUs (72). Some methods employing molecular biology can detect mixed T. cruzi infections, such as those used in the protocol proposed by D´Ávilla et al. (2009) (228) (114, 409). Andrade et al. (2011) (114) used the PCR-RFLP technique of the subunit II cytochrome oxidase gene and analyzed the polymorphism of the 24sα-LSU rDNA gene, as well as the polymorphism of mini-exon SL-IL intergenic spacer, to demonstrate mixed infections with TcI + TcIII and TcII + TcVI DTUs of T. cruzi in parasites isolated from patients with acute Chagas disease, triatomines, and wild reservoirs. Sá et al. (2016) (409) also verified mixed T. cruzi infections in the intestinal content of Rhodinus prolixus using the criteria of D´Ávilla et al. (2009) (228). The researchers identified 79.4% of mixed T. cruzi infections; however, PCR-RFLP of the subunit II cytochrome oxidase gene was not able to recognize mixed infections by TcV+ TcVI, requiring association of this technique with the analyses of the 24sα-LSU rDNA gene polymorphism. Previous studies have shown mixed T. cruzi infections by kDNA PCR amplification and subsequent hybridization using DTU-specific probes (DTU-blot) (354, 410), PCR-Real Time with Taq-Man probes (MTq-PCR) (400), and the amplification of other parasite genome targets, such as the cSC5D gene by PCR-RFL (384). Moreover, some studies revealed that parasite populations in blood hosts were distinct from those isolated by xenodiagnosis and grown in culture media (353, 354, 410). Ortiz et al. (2015) (410) demonstrated mixtures of TcI, TcII, TcV, and TcVI in blood samples of patients with chronic Chagas disease via kDNA PCR amplification and subsequent hybridization with DTU-specific probe, while in the xenodiagnosis followed by axenic culture, most parasites were identified with TcV. Even when using different targets from the T. cruzi genome, studies such as Consentino and Aguero (2012) (384) and Cura et al. (2015) (400) did not detect all mixed parasite infections in biological samples. Consentino and Aguero (2012) (384), through the PCR-RFLP of the cSC5D gene, did not identify mixed infections from the TcV + TcVI T. cruzi DTUs. Cura et al. (2015) (400) were unable to verify the mixed infection from TcI + TcII/TcV/TcVI DTUs in patients with Chagas disease by the PCR-Real Time with Taq-Man probes (MTq-PCR) and failed to detect mixed T. cruzi infections in triatomine and wild reservoir samples. Nevertheless, even using high-complexity methodologies, some approaches could not detect all the mixed infections in hosts infected with T. cruzi.
The molecular methods used for the genotype-specific diagnosis of Chagas disease have advantages and drawbacks, such as high complexity, extended analysis time, and misleading interpretation of the results, and all of them are based on genetic signatures (411). The genotyping of T. cruzi infections in chagasic chronic hosts by these methodologies still represents a challenge, as some of these methods require several genetic markers to distinguish T. cruzi genotypes (6, 22, 67, 228, 276). Furthermore, the majority of biochemical/molecular approaches cannot be directly performed in biological and clinical samples, requiring previous parasite isolation by low-sensitivity methods (hemoculture or xenodiagnosis) followed by in vitro growth, which may lead to clonal selection (61, 125, 220, 410, 412–414). In addition, according to the Clonal Histiotropic Model, parasite isolates from peripheral blood samples do not necessarily represent the complete set of T. cruzi DTUs found in distinct host tissues (126, 127, 221, 234). Parasitemia in patients and reservoirs infected with T. cruzi is variable, and the success of parasite isolation is dependent on host parasitemia; therefore, several strategies proposed to genotype T. cruzi isolates have been applied only to cultured stocks and biological or clinical samples with high parasitic loads (47, 118, 276, 343, 384, 400, 415, 416). Another limitation is that target sequences of T. cruzi amplified by molecular methods for DTU identification are found in a single copy number or low copy numbers; therefore, the use of targets with high copy numbers, such as SatDNA and kDNA mHVR sequences, has a significant impact in clinical and epidemiological studies (69, 70, 403, 407). Given these limitations, further research is required to optimize the sensitivity and simplify methods for genotyping T. cruzi DTUs that can be easily applied in clinical laboratories (407, 417).
Previous studies have shown the influence of T. cruzi genotype on the antibody response pattern in experimental models (88, 418, 419). The profile of lytic antibodies varies when distinct T. cruzi strains are used as target antigens, suggesting that genotype-specific antigenic features of the parasite could be involved in the induction of lytic antibodies (418–420). Dos Santos et al. (2009) (88) correlated T. cruzi genetic diversity with the pattern of humoral immune responses in mice infected with different parasite genotypes.
Several innovative serological methods have been developed to overcome the limitations of molecular tests for genotype-specific diagnosis of Chagas disease, mainly during the chronic phase (362–371, 421). The first serological marker to identify T. cruzi genotypes was the mucin trypomastigote small surface antigen (TSSA), a surface protein of the bloodstream trypomastigote, which initially appeared to correlate with the parasite lineages T. cruzi I (TcI) and T. cruzi II (TcII) (422). Bhattacharyya et al. (2010, 2014, 2015) (362, 364, 365) verified that TSSA pepII/V/VI isoforms could distinguish samples of hosts infected with different T. cruzi genotypes. Bhattacharyya et al. (2010) (362) identified TSSA II as an antigen capable of recognizing TcII, TcV, and TcVI T. cruzi DTUs as distinct from infections by TcI, TcIII, and TcIV DTUs; already the peptide described specific for TcI infections (TSSA I) share some characteristics with as TcIII and TcIV DTUs. The researchers found greater T. cruzi lineage-specific diversity than that previously described by Di Noia et al. (2002) (422). Bhattacharyya et al. (2014) (364) evaluated the reactivity of serum samples from infected mice with different T. cruzi genotypes and human samples from distinct geographical regions of Latin America by ELISA using the TSSA antigen. The results obtained in this study showed that sera from mice infected with TcII, TcV, or TcVI DTUs recognized the TSSApep-II/V/VI antigen, whereas sera from mice infected with TcIII or TcIV DTUs reacted with their respective TSSA peptides. In this work, the sera of patients with Chagas disease from the Southern Cone reacted with TSSApep-II/V/VI and TSSApep-V/VI antigens; however, the samples of patients with Chagas disease from the Amazon North region did not specifically identify the TcI antigen. Bhattacharyya et al. (2010, 2014, 2015) (362, 364, 365) indicated that the protein core of TSSA contains remarkable polymorphism, in which TcI, TcIII, and TcIV have their lineage-specific sequences; TcII, TcV, and TcVI share a common epitope, and the hybrid lineages TcV and TcVI share an additional epitope. In this context, the authors proposed that TSSA isoforms are feasible serological markers for identifying T. cruzi DTUs in humans and experimental infections. Nevertheless, TSSApep-I did not show significant reactivity with human chagasic sera from TcI-endemic regions, potentially due to low immunogenicity or a conformation of the peptide differing from that of the native antigen, suggesting that novel targets for TcI are still required (362, 364, 365). Ker et al. (2016) (366) applied ELISA using lineage-specific TSSA epitopes to the sera of Brazilian primates from the Atlantic Forest and Amazon regions to identify T. cruzi DTUs that infect hosts and further expand the knowledge of transmission cycles in these biomes. This study demonstrated that both peptides, TSSApep-II/V/VI and TSSApep-V/VI, gave lineage-specific reactions with samples from the Atlantic Forest; conversely, only one Amazonian primate produced a peptide reaction with only TSSApep-III and TSSApep-IV. The authors concluded that this novel methodology readily applies to many mammalian species. However, for the discovery of T. cruzi genotype, more attempts should be made to design an efficacious TcI-specific peptide. Bhattacharyya et al. (2018) (369) developed a rapid diagnostic test (RDT; Chagas Sero K-SeT) that incorporates a peptide that corresponds to the TSSA II/V/VI common epitope and tested it with sera of patients with Chagas disease from Bolivia and Peru, including individuals with varying cardiac pathology, in addition to matched mothers and neonates. This study showed that Chagas Sero K-SeT RDT can replace ELISA for TSSApep-II/V/VI serology and that the response to this RDT was associated with the severity of Chagas cardiomyopathy in Bolivian patients and thus may have prognostic value. Chagas Sero K-SeT RDT using the TSSApep-II/V/VI common epitope peptide and TSSApep ELISA were applied for T. cruzi lineage-specific diagnosis in serum samples from humans and mammals in the Chaco region of northern Argentina to gain further insight into ecological and epidemiological associations (423). Chagas Sero K-SeT RDT detected human and canine IgG and was as sensitive as TSSApep-II/V/VI ELISA. Moreover, seropositivity by both methods was 69.5% for humans, 65.8% for dogs, and 14.3% for armadillos; by ELISA, it was 26.3% for cats (423). Another study used the Chagas Sero K-SeT RDT, which incorporates the TSSApep-II/V/VI peptide epitope for T. cruzi lineage-specific diagnosis in serum samples from experimentally infected murine and sera from a range of mammalian orders and biomes in Brazil for epidemiological surveillance (421). This method identified the TcII/V/VI genotypes in experimental murine T. cruzi infections and natural infections across several orders of Brazilian mammals, namely, Primata, Carnivora (canine), Rodentia, and Chiroptera; however, Chagas Sero K-SeT RDT could not recognize IgG from felines and armadillos (421). The Chagas Sero K-SeT RDT is directly applicable to TcII/V/VI-specific serological surveillance of T. cruzi infection among potential animal reservoirs of the parasite, to assess the risk of emergent endemic regions and to guide control strategies without the need to isolate T. cruzi from the animals (421, 423). Other T. cruzi target antigens have been investigated by Mendes et al. (2013) (363) for application in the ELISA test for genotype-specific diagnosis of Chagas disease. Mendes et al. (2013) (363) identified three polymorphic linear B-cell epitopes on proteins derived from a pair of alleles of the hybrid CL Brener strain genome that could be used for serotyping Chagas disease by ELISA. These authors tested serum samples from mice infected with Colombian (TcI), CL (TcVI), and Y (TcII), as well as sera from patients with Chagas disease infected with TcI and TcII DTUs. This study demonstrated that three polymorphic epitopes could discriminate TcI and TcII infections; however, samples from animals infected with TcVI showed cross-reactivity with the peptides. This study demonstrated the potential of these target antigens for Chagas disease serotyping, but it is necessary to improve antigen search and develop a robust panel of strain-specific epitopes to achieve a method applicable to large epidemiological studies.
Previous studies have developed a new serological method for improving genotype-specific diagnosis of Chagas disease to provide the profile of T. cruzi DTUs that infect the patients (367–369). A flow cytometry-based technique, called Chagas-Flow ATE (Amastigote, Trypomastigote, and Epimastigote), was developed to assess the expression of anti-amastigote, anti-trypomastigote, and anti-epimastigote antibodies, displaying high performance for the diagnosis and post-therapeutic monitoring of Chagas disease (357). Subsequently, Alessio et al. (2017) (367) optimized the Chagas-Flow ATE for genotype-specific diagnosis of a single experimental T. cruzi infection in the chronic phase, using as target antigens parallel batches of distinct T. cruzi genotypes representative of three major DTUs involved mainly in the domestic (TcI, TcII, and TcVI) and sylvatic (TcI) cycles of Chagas disease in Brazil (4, 5). The researchers demonstrated that the Chagas-Flow ATE methodology showed 74% global accuracy in discriminating infections with TcI vs. TcVI vs. TcII DTUs and 94% global accuracy in segregating infections with TcI vs. TcII, showing its applicability for genotype-specific diagnosis of single experimental T. cruzi infection in the chronic phase. To extend the early studies carried out by Alessio et al. (2017) (367), the same research group evaluated the performance of the Chagas-Flow ATE methodology for early and late differential diagnosis of single and dual genotype-specific experimental T. cruzi infections (368). Alessio et al. (2018) (368) revealed that during the early stage, this new method presented 72% global accuracy in differentiating single infections and 80% global accuracy in segregating T. cruzi dual infections, while in the late stage, the global accuracy was 69% for discriminating single infections and 76% for T. cruzi dual infections. The authors concluded that Chagas-Flow ATE showed good performance for genotype-specific diagnosis of single and dual experimental T. cruzi infections, in both early and late stages, indicating that this technique presented an accuracy of 81% as a complementary diagnostic test for T. cruzi infection (368). Alessio et al. (2020) (371) evaluated the performance of Chagas-Flow ATE for DTU-specific diagnosis of Chagas disease using serum samples from patients with Chagas disease chronically infected with TcI, TcVI, or TcII DTUs of T. cruzi. The results obtained in this work demonstrated the high performance of the Chagas-Flow ATE technique for DTU-specific diagnosis of Chagas disease, with 92% global accuracy in discriminating “TcI vs. TcVI vs. TcII” and 97% global accuracy in segregating “TcI vs. TcII” T. cruzi infections. Earlier studies were pioneers in the development of a serological method by flow cytometry for the genotype-specific diagnosis of Chagas disease, with advantages such as the use of a wide range of antigenic preparations in a single flow cytometric platform (357, 424–427), testing of a large number of samples, and evaluation of the reactivity of serum samples from single and mixed T. cruzi infections (367, 368, 371).
Innovative work proposed a method based on mass spectrometry for genotyping T. cruzi DTUs (411). T. cruzi revealed differential protein expression in distinct genetic groups of parasites (411). De Oliveira et al. (2018) (411) developed a T. cruzi Strain Typing Assay using MS2 peptide spectral libraries (Tc-STAMS2). The method was based on constructing a spectra library, which was inspected using MS/MS spectra from unknown T. cruzi strains and assigned to a specific strain in an automated and computationally driven approach. Therefore, using shotgun proteomics combined with spectral library search, T. cruzi strains can be discriminated independently from genome knowledge. The Tc-STAMS2 method identified more than 4,000 proteins in the combined six DTUs strains, and 1,096 proteins were differentially expressed between the six DTUs; multivariate analysis allowed the discrimination of T. cruzi strains using the quantitative MS signal. The authors concluded that Tc-STAMS2 represents a complementary strategy for genotyping T. cruzi DTUs using only fragmentation spectra without needing genomic data (411).
The identification of new biomarkers for use in T. cruzi genotyping is a current research challenge (417). A primary focus in Chagas disease studies is establishing an association between DTUs and clinical manifestations of the disease or response to treatment (417). Therefore, methods of discerning single and mixed T. cruzi infections during diagnosis are paramount. This capability directly impacts predicting disease morbidity, assessing the response to chemotherapy, and anticipating reactivation in immunocompromised patients (65, 402, 428). Furthermore, the notable genetic diversity of T. cruzi, coupled with the distinct geographic distribution and transmission cycles of DTUs, elevates the significance of their identification. This aspect is crucial for ecological and epidemiological surveillance, laying the groundwork for precise disease control strategies (5, 7, 20).
10 Discussion
The clinical outcome of Chagas disease is influenced by several factors, among which the most important are the host’s immune system and the biological and genetic characteristics of the parasite. Several studies have demonstrated that the genetic variability of T. cruzi directly influences its infectivity and virulence. These factors, which are closely linked to the epidemiology and immunopathogenesis of the disease, play an important role in the response of infected individuals and the evolution of Chagas disease. This study aimed to describe some aspects of T. cruzi genetic diversity, its interaction with the host, and the principal methodologies used for genotyping the parasite. In this context, several methodologies are available for genotype-specific diagnosis of Chagas disease, including molecular methods. Each of these approaches has its advantages and drawbacks, so the choice of which should be used in T. cruzi genotyping must take into account aspects such as the type of sample, the presence of mixed parasite infections, the genetic marker, and the process of DNA or RNA extraction (in the molecular methods). The geographic distribution of the different DTUs of T. cruzi can influence the parasite’s transmission cycle, the host’s adaptability, treatment response, and the infected individuals’ clinical course.
The genetic variability of T. cruzi plays a crucial role in parasite infectivity, reproduction, and differentiation within vectors. Some strains may exhibit distinct distributions among hosts, signifying preferential tissue tropism, particularly in T. cruzi mixed infections. Conversely, studies indicating a lack of correlation between the clinical manifestations of Chagas disease and T. cruzi DTUs should be considered. The genetic makeup of T. cruzi is directly associated with disease reactivation events and symptoms in immunosuppressed patients. Although genetic variability significantly contributes to the pathogenesis of the disease, further investigations are imperative to comprehensively assess these aspects, particularly within the context of mixed infections and reinfections. In this milieu, strains comprising different T. cruzi DTUs and distinct populations involved in infection have the potential to modulate the immune response, thereby influencing the pathogenesis of the disease and response to treatment. Evaluation of intragroup variability and parasite gene expression should be conducted more comprehensively to enhance our understanding of the impact of this parameter.
It is currently difficult to determine a direct association between the genetics of T. cruzi and the development of different clinical conditions in Chagas disease. However, identifying the different immunological and pathogenic mechanisms triggered by different strains can provide important information for better monitoring affected patients and directing possible therapies. Knowledge of the genetic variability of T. cruzi and its countless implications in the course of disease in infected individuals may contribute to strategies for control, clinical prognosis status of ongoing infection, and post-therapeutic monitoring of the disease. Thus, it is necessary to carry out studies that address in an integrated manner all aspects related to the genetic variability of T. cruzi and their implications in the different outcomes of Chagas disease.
Author contributions
MM: Writing – review & editing, Writing – original draft, Methodology, Investigation, Conceptualization. GA: Writing – review & editing, Writing – original draft, Methodology, Investigation, Conceptualization. BF: Writing – original draft, Methodology, Investigation, Conceptualization. PS: Writing – review & editing, Methodology, Investigation, Data curation, Conceptualization. MA: Writing – review & editing, Methodology, Investigation, Data curation, Conceptualization. CS: Writing – review & editing, Methodology. GB: Writing – review & editing, Methodology, Investigation, Data curation, Conceptualization. OM-F: Writing – review & editing, Supervision, Methodology, Investigation, Funding acquisition, Data curation, Conceptualization. AT-C: Writing – review & editing, Supervision, Project administration, Methodology, Investigation, Funding acquisition, Data curation, Conceptualization. HM: Writing – review & editing, Writing – original draft, Supervision, Project administration, Methodology, Investigation, Funding acquisition, Data curation, Conceptualization.
Funding
The author(s) declare financial support was received for the research, authorship, and/or publication of this article. This work was supported by Fundação de Amparo à Pesquisa do Estado de Minas Gerais (FAPEMIG) (Process APQ-02434-16), Conselho Nacional de Desenvolvimento Científico e Tecnológico (CNPq) and Coordenação de Aperfeiçoamento de Pessoal de Nível Superior (CAPES). The authors thank the Integrated Center for Postgraduate Studies and Research in Health at UFVJM, Diamantina, MG; Pharmacy Department and Centro René Rachou. ATC received PQ fellowships from CNPq.
Acknowledgments
OAMF is research fellow from Universidade do Estado do Amazonas-UEA (PROVIST No. 005/2023-PROPESP/UEA). We thank Liliane Martins dos Santos for scientific discussions.
Conflict of interest
The authors declare that the research was conducted in the absence of any commercial or financial relationships that could be construed as a potential conflict of interest.
Publisher’s note
All claims expressed in this article are solely those of the authors and do not necessarily represent those of their affiliated organizations, or those of the publisher, the editors and the reviewers. Any product that may be evaluated in this article, or claim that may be made by its manufacturer, is not guaranteed or endorsed by the publisher.
References
1. Chagas C. Nova Tripanozomiase Humana. Estudo sobre a morfologia e o ciclo evolutivo do Schizotrypanum cruzi. n. gen., n. sp. agente etiológico de nova entidade mórbida do homem. Mem Inst Oswaldo Cruz. (1909) 1:159–218.
2. Dias JC, Ramos AN Jr, Gontijo ED, Luquetti A, Shikanai-Yasuda MA, Coura JR, et al. II Consenso Brasileiro em Doença de Chagas, 2015 [Brazilian Consensus on Chagas Disease, 2015]. Epidemiol Serv Saude. (2016) 25:7–86. doi: 10.5123/S1679-49742016000500002
3. World Health Organization. Chagas disease (American trypanosomiasis) (2022). Available online at: https://www.who.int/campaigns/world-chagas-disease-day/2022.
4. Tibayrenc M, Ayala FJ. The clonal theory of parasitic protozoa: 12 years on. Trends Parasitol. (2002) 18:405–10. doi: 10.1016/s1471-4922(02)02357-7
5. Zingales B, Miles MA, Campbell DA, Tibayrenc M, Macedo AM, Teixeira MM, et al. The revised Trypanosoma cruzi subspecific nomenclature: rationale, epidemiological relevance and research applications. Infect Genet Evol. (2012) 12:240–53. doi: 10.1016/j.meegid.2011.12.009
6. Lewis MD, Ma J, Yeo M, Carrasco HJ, Llewellyn MS, Miles MA. Genotyping of Trypanosoma cruzi: systematic selection of assays allowing rapid and accurate discrimination of all known lineages. Am J Trop Med Hyg. (2009) 81:1041–9. doi: 10.4269/ajtmh.2009.09-0305
7. Zingales B. Trypanosoma cruzi genetic diversity: Something new for something known about Chagas disease manifestations, serodiagnosis and drug sensitivity. Acta Trop. (2018) 184:38–52. doi: 10.1016/j.actatropica.2017.09.017
8. Miles MA, Toye PJ, Oswald SC, Godfrey DG. The identification by isoenzyme patterns of two distinct strain-groups of Trypanosoma cruzi, circulating independently in a rural area of Brazil. Trans R Soc Trop Med Hyg. (1977) 71:217–25. doi: 10.1016/0035-9203(77)90012-8
9. Miles MA, Souza A, Povoa M, Shaw JJ, Lainson R, Toye PJ. Isozymic heterogeneity of Trypanosoma cruzi in the first autochthonous patients with Chagas’ disease in Amazonian Brazil. Nature. (1978) 272:819–21. doi: 10.1038/272819a0
10. Romanha AJ, da Silva Pereira AA, Chiari E, Kilgour V. Isoenzyme patterns of cultured Trypanosoma cruzi: changes after prolonged subculture. Comp Biochem Physiol B. (1979) 62:139–42. doi: 10.1016/0305-0491(79)90299-2
11. Morel C, Chiari E, Camargo EP, Mattei DM, Romanha AJ, Simpson L. Strains and clones of Trypanosoma cruzi can be characterized by pattern of restriction endonuclease products of kinetoplast DNA minicircles. Proc Natl Acad Sci U.S.A. (1980) 77:6810–4. doi: 10.1073/pnas.77.11.6810
12. Andrade SG. Caracterização de cepas de Trypanosoma cruzi isoladas no Recôncavo Baiano. Rev.Pat.Trop. (1974) 3:65–121.
13. Andrade LO, Galvão LM, Meirelles Mde N, Chiari E, Pena SD, Macedo AM. Differential tissue tropism of Trypanosoma cruzi strains: an in vitro study. Mem Inst Oswaldo Cruz. (2010) 105:834–7. doi: 10.1590/s0074-02762010000600018
14. Tibayrenc M, Ayala FJ. Towards a population genetics of microorganisms: The clonal theory of parasitic protozoa. Parasitol Today. (1991) 7:228–32. doi: 10.1016/0169-4758(91)90234-f
15. Tibayrenc M. Population genetics of parasitic protozoa and other microorganisms. Adv Parasitol. (1995) 36:47–115. doi: 10.1016/s0065-308x(08)60490-x
16. Souto RP, Fernandes O, Macedo AM, Campbell DA, Zingales B. DNA markers define two major phylogenetic lineages of Trypanosoma cruzi. Mol Biochem Parasitol. (1996) 83:141–52. doi: 10.1016/s0166-6851(96)02755-7
17. Kawashita SY, Sanson GF, Fernandes O, Zingales B, Briones MR. Maximum-likelihood divergence date estimates based on rRNA gene sequences suggest two scenarios of Trypanosoma cruzi intraspecific evolution. Mol Biol Evol. (2001) 18:2250–9. doi: 10.1093/oxfordjournals.molbev.a003771
18. Tibayrenc M. Integrated genetic epidemiology of infectious diseases: the Chagas model. Mem Inst Oswaldo Cruz. (1998) 93:577–80. doi: 10.1590/s0074-02761998000500003
19. Anonymous. Recommendations from a satellite meeting. Mem Inst Oswaldo Cruz. (1999) 1:429–32. doi: 10.1590/s0074-02761999000700085
20. Miles MA, Llewellyn MS, Lewis MD, Yeo M, Baleela R, Fitzpatrick S, et al. The molecular epidemiology and phylogeography of Trypanosoma cruzi and parallel research on Leishmania: looking back and to the future. Parasitology. (2009) 136:1509–28. doi: 10.1017/S0031182009990977
21. Brisse S, Barnabé C, Tibayrenc M. Identification of six Trypanosoma cruzi phylogenetic lineages by random amplified polymorphic DNA and multilocus enzyme electrophoresis. Int J Parasitol. (2000) 30:35–44. doi: 10.1016/s0020-7519(99)00168-x
22. Brisse S, Verhoef J, Tibayrenc M. Characterisation of large and small subunit rRNA and mini-exon genes further supports the distinction of six Trypanosoma cruzi lineages. Int J Parasitol. (2001) 31:1218–26. doi: 10.1016/s0020-7519(01)00238-7
23. Robello C, Gamarro F, Castanys S, Alvarez-Valin F. Evolutionary relationships in Trypanosoma cruzi: molecular phylogenetics supports the existence of a new major lineage of strains. Gene. (2000) 246:331–8. doi: 10.1016/s0378-1119(00)00074-3
24. Augusto-Pinto L, Teixeira SM, Pena SD, MaChado CR. Single-nucleotide polymorphisms of the Trypanosoma cruzi MSH2 gene support the existence of three phylogenetic lineages presenting differences in mismatch-repair efficiency. Genetics. (2003) 164:117–26. doi: 10.1093/genetics/164.1.117
25. Henriksson J, Dujardin JC, Barnabé C, Brisse S, Timperman G, Venegas J, et al. Chromosomal size variation in Trypanosoma cruzi is mainly progressive and is evolutionarily informative. Parasitology. (2002) 124:277–86. doi: 10.1017/s0031182001001093
26. Freitas JM, Augusto-Pinto L, Pimenta JR, Bastos-Rodrigues L, Gonçalves VF, Teixeira SM, et al. Ancestral genomes, sex, and the population structure of Trypanosoma cruzi. PloS Pathog. (2006) 2:e24. doi: 10.1371/journal.ppat.0020024
27. Rozas M, Botto-Mahan C, Coronado X, Ortiz S, Cattan PE, Solari A. Coexistence of Trypanosoma cruzi genotypes in wild and periodomestic mammals in Chile. Am J Trop Med Hyg. (2007) 77:647–53.
28. Perez-Ramirez L, Barnabé C, Sartori AM, Ferreira MS, Tolezano JE, Nunes EV, et al. Clinical analysis and parasite genetic diversity in human immunodeficiency virus/Chagas’ disease coinfections in Brazil. Am J Trop Med Hyg. (1999) 61:198–206. doi: 10.4269/ajtmh.1999.61.198
29. Vicco MH, Ferini F, Rodeles L, Cardona P, Bontempi I, Lioi S, et al. Assessment of cross-reactive host-pathogen antibodies in patients with different stages of chronic Chagas disease. Rev Esp Cardiol (Engl Ed). (2013) 66(10):791–6. doi: 10.1016/j.rec.2013.05.028
30. Rodriguez HO, Guerrero NA, Fortes A, Santi-Rocca J, Gironès N, Fresno M. Trypanosoma cruzi strains cause different myocarditis patterns in infected mice. Acta Trop. (2014) 139:57–66. doi: 10.1016/j.actatropica.2014.07.005
31. Poveda C, Fresno M, Gironès N, Martins-Filho OA, Ramírez JD, Santi-Rocca J, et al. Cytokine profiling in Chagas disease: towards understanding the association with infecting Trypanosoma cruzi discrete typing units (a BENEFIT TRIAL sub-study). PloS One. (2014) 9:e91154. doi: 10.1371/journal.pone.0091154
32. Prata A. Clinical and epidemiological aspects of Chagas disease. Lancet Infect Dis. (2001) 1:92–100. doi: 10.1016/S1473-3099(01)00065-2
33. Rassi A Jr, Rassi A, Marin-Neto JA. Chagas heart disease: pathophysiologic mechanisms, prognostic factors and risk stratification. Mem Inst Oswaldo Cruz. (2009) 104 Suppl 1:152–8. doi: 10.1590/s0074-02762009000900021
34. Vitelli-Avelar DM, Sathler-Avelar R, Mattoso-Barbosa AM, Gouin N, Perdigão-de-Oliveira M, Valério-Dos-Reis L, et al. Cynomolgus macaques naturally infected with Trypanosoma cruzi-I exhibit an overall mixed pro-inflammatory/modulated cytokine signature characteristic of human Chagas disease. PloS Negl Trop Dis. (2017) 11(2):e0005233. doi: 10.1371/journal.pntd.0005233
35. Guedes PM, Gutierrez FR, Silva GK, Dellalibera-Joviliano R, Rodrigues GJ, Bendhack LM, et al. Deficient regulatory T cell activity and low frequency of IL-17-producing T cells correlate with the extent of cardiomyopathy in human Chagas’ disease. PloS Negl Trop Dis. (2012) 6:e1630. doi: 10.1371/journal.pntd.0001630
36. Ramírez JD, Hernández C, Montilla M, Zambrano P, Flórez AC, Parra E, et al. First report of human Trypanosoma cruzi infection attributed to TcBat genotype. Zoonoses Public Health. (2014) 61:477–9. doi: 10.1111/zph.12094
37. Jiménez P, Jaimes J, Poveda C, Ramírez JD. A systematic review of the Trypanosoma cruzi genetic heterogeneity, host immune response and genetic factors as plausible drivers of chronic chagasic cardiomyopathy. Parasitology. (2019) 146(3):269–83. doi: 10.1017/S0031182018001506
38. Zingales B, Andrade SG, Briones MR, Campbell DA, Chiari E, Fernandes O, et al. A new consensus for Trypanosoma cruzi intraspecific nomenclature: second revision meeting recommends TcI to TcVI. Mem Inst Oswaldo Cruz. (2009) 104:1051–4. doi: 10.1590/s0074-02762009000700021
39. Marcili A, Lima L, Valente VC, Valente SA, Batista JS, Junqueira AC, et al. Comparative phylogeography of Trypanosoma cruzi TCIIc: new hosts, association with terrestrial ecotopes, and spatial clustering. Infect Genet Evol. (2009) 9:1265–74. doi: 10.1016/j.meegid.2009.07.003
40. Lima L, Espinosa-Álvarez O, Ortiz PA, Trejo-Varón JA, Carranza JC, Pinto CM, et al. Genetic diversity of Trypanosoma cruzi in bats, and multilocus phylogenetic and phylogeographical analyses supporting Tcbat as an independent DTU (discrete typing unit). Acta Trop. (2015) 151:166–77. doi: 10.1016/j.actatropica.2015.07.015
41. Guhl F, Ramírez JD. Retrospective molecular integrated epidemiology of Chagas disease in Colombia. Infect Genet Evol. (2013) 20:148–54. doi: 10.1016/j.meegid.2013.08.028
42. Barnabé C, Mobarec HI, Jurado MR, Cortez JA, Brenière SF. Reconsideration of the seven discrete typing units within the species Trypanosoma cruzi, a new proposal of three reliable mitochondrial clades. Infect Genet Evol. (2016) 39:176–86. doi: 10.1016/j.meegid.2016.01.029
43. Westenberger SJ, Barnabé C, Campbell DA, Sturm NR. Two hybridization events define the population structure of Trypanosoma cruzi. Genetics. (2005) 171:527–43. doi: 10.1534/genetics.104.038745
44. Westenberger SJ, Cerqueira GC, El-Sayed NM, Zingales B, Campbell DA, Sturm NR. Trypanosoma cruzi mitochondrial maxicircles display species- and strain-specific variation and a conserved element in the non-coding region. BMC Genomics. (2006) 7:60. doi: 10.1186/1471-2164-7-60
45. Sturm NR, Campbell DA. Alternative lifestyles: the population structure of Trypanosoma cruzi. Acta Trop. (2010) 115:35–43. doi: 10.1016/j.actatropica.2009.08.018
46. Subileau M, Barnabé C, Douzery EJ, Diosque P, Tibayrenc M. Trypanosoma cruzi: new insights on ecophylogeny and hybridization by multigene sequencing of three nuclear and one maxicircle genes. Exp Parasitol. (2009) 122:328–37. doi: 10.1016/j.exppara.2009.04.008
47. Yeo M, Mauricio IL, Messenger LA, Lewis MD, Llewellyn MS, Acosta N, et al. Multilocus sequence typing (MLST) for lineage assignment and high resolution diversity studies in Trypanosoma cruzi. PloS Negl Trop Dis. (2011) 5:e1049. doi: 10.1371/journal.pntd.0001049
48. Lewis MD, Llewellyn MS, Yeo M, Acosta N, Gaunt MW, Miles MA. Recent, independent and anthropogenic origins of Trypanosoma cruzi hybrids. PloS Negl Trop Dis. (2011) 5:e1363. doi: 10.1371/journal.pntd.0001363
49. Zingales B, Bartholomeu DC. Trypanosoma cruzi genetic diversity: impact on transmission cycles and Chagas disease. Mem Inst Oswaldo Cruz. (2022) 117:e210193. doi: 10.1590/0074-02760210193
50. Dumonteil E, Elmayan A, Majeau A, Tu W, Duhon B, Marx P, et al. Genetic diversity of Trypanosoma cruzi parasites infecting dogs in southern Louisiana sheds light on parasite transmission cycles and serological diagnostic performance. PloS Negl Trop Dis. (2020) 14:e0008932. doi: 10.1371/journal.pntd.0008932
51. Rassi A Jr, Rassi A, Marin-Neto JA. Chagas disease. Lancet. (2010) 375:1388–402. doi: 10.1016/S0140-6736(10)60061-X
52. Pérez-Molina JA, Molina I. Chagas disease. Lancet. (2018) 391:82–94. doi: 10.1016/S0140-6736(17)31612-4
53. World Health Organization. Chagas disease. (2020). Available online at: https://www.who.int/health-topics/chagas-disease#tab=tab_1.
54. Vaidian AK, Weiss LM, Tanowitz HB. Chagas’ disease and AIDS. Kinetoplastid Biol Dis. (2004) 3:2. doi: 10.1186/1475-9292-3-2
55. Pinazo MJ, Espinosa G, Cortes-Lletget C, Posada Ede J, Aldasoro E, Oliveira I, et al. Immunosuppression and Chagas disease: a management challenge. PloS Negl Trop Dis. (2013) 7:e1965. doi: 10.1371/journal.pntd.0001965
56. Suárez C, Nolder D, García-Mingo A, Moore DAJ, Chiodini PL. Diagnosis and clinical management of chagas disease: an increasing challenge in non-endemic areas. Res Rep Trop Med. (2022) 13:25–40. doi: 10.2147/RRTM.S278135
57. Crowder LA, Wendel S, Bloch EM, O’Brien SF, Delage G, Sauleda S, et al. WP-TTID Subgroup on Parasites. International survey of strategies to mitigate transfusion-transmitted Trypanosoma cruzi in non-endemic countries, 2016-2018. Vox Sang. (2022) 117:58–63. doi: 10.1111/vox.13164
58. Miles MA, Cedillos RA, Póvoa MM, de Souza AA, Prata A, Macedo V. Do radically dissimilar Trypanosoma cruzi strains (zymodemes) cause Venezuelan and Brazilian forms of Chagas’ disease? Lancet. (1981) 1:1338–40. doi: 10.1016/s0140-6736(81)92518-6
59. Coura JR, Junqueira AC, Fernandes O, Valente SA, Miles MA. Emerging chagas disease in amazonian Brazil. Trends Parasitol. (2002) 18:171–6. doi: 10.1016/s1471-4922(01)02200-0
60. Buscaglia CA, Di Noia JM. Trypanosoma cruzi clonal diversity and the epidemiology of Chagas’ disease. Microbes Infect. (2003) 5:419–27. doi: 10.1016/s1286-4579(03)00050-9
61. Burgos JM, Diez M, Vigliano C, Bisio M, Risso M, Duffy T, et al. Molecular identification of Trypanosoma cruzi discrete typing units in end-stage chronic Chagas heart disease and reactivation after heart transplantation. Clin Infect Dis. (2010) 51:485–95. doi: 10.1086/655680
62. Monteiro WM, Magalhães LK, de Sá AR, Gomes ML, Toledo MJ, Borges L, et al. Trypanosoma cruzi IV causing outbreaks of acute Chagas disease and infections by different haplotypes in the Western Brazilian Amazonia. PloS One. (2012) 7:e41284. doi: 10.1371/journal.pone.0041284
63. Carranza JC, Valadares HM, D’Avila DA, Baptista RP, Moreno M, Galvão LM, et al. Trypanosoma cruzi maxicircle heterogeneity in Chagas disease patients from Brazil. Int J Parasitol. (2009) 39:963–73. doi: 10.1016/j.ijpara.2009.01.009
64. del Puerto R, Nishizawa JE, Kikuchi M, Iihoshi N, Roca Y, Avilas C, et al. Lineage analysis of circulating Trypanosoma cruzi parasites and their association with clinical forms of Chagas disease in Bolivia. PloS Negl Trop Dis. (2010) 4:e687. doi: 10.1371/journal.pntd.0000687
65. Coura JR, Junqueira AC. Risks of endemicity, morbidity and perspectives regarding the control of Chagas disease in the Amazon Region. Mem Inst Oswaldo Cruz. (2012) 107:145–54. doi: 10.1590/s0074-02762012000200001
66. Souza RCM, Gorla DE, Chame M, Jaramillo N, Monroy C, Diotaiuti L. Chagas disease in the context of the 2030 agenda: global warming and vectors. Mem Inst Oswaldo Cruz. (2022) 117:e200479. doi: 10.1590/0074-02760200479
67. de Oliveira MT, de Assis GF, Oliveira e Silva JC, MaChado EM, da Silva GN, Veloso VM, et al. Trypanosoma cruzi Discret Typing Units (TcII and TcVI) in samples of patients from two municipalities of the Jequitinhonha Valley, MG, Brazil, using two molecular typing strategies. Parasit Vectors. (2015) 8:568. doi: 10.1186/s13071-015-1161-2
68. Herrera CP, Licon MH, Nation CS, Jameson SB, Wesson DM. Genotype diversity of Trypanosoma cruzi in small rodents and Triatoma sanguisuga from a rural area in New Orleans, Louisiana. Parasit Vectors. (2015) 8:123. doi: 10.1186/s13071-015-0730-8
69. Hernández C, Cucunubá Z, Flórez C, Olivera M, Valencia C, Zambrano P, et al. Molecular diagnosis of chagas disease in Colombia: parasitic loads and discrete typing units in patients from acute and chronic phases. PloS Negl Trop Dis. (2016) 10:e0004997. doi: 10.1371/journal.pntd.0004997
70. Ramírez JD, Guhl F, Rendón LM, Rosas F, Marin-Neto JA, Morillo CA. Chagas cardiomyopathy manifestations and Trypanosoma cruzi genotypes circulating in chronic Chagasic patients. PloS Negl Trop Dis. (2010) 4:e899. doi: 10.1371/journal.pntd.0000899
71. Enriquez GF, Cardinal MV, Orozco MM, Schijman AG, Gürtler RE. Detection of Trypanosoma cruzi infection in naturally infected dogs and cats using serological, parasitological and molecular methods. Acta Trop. (2013) 126:211–7. doi: 10.1016/j.actatropica.2013.03.001
72. Monje-Rumi MM, Brandán CP, Ragone PG, Tomasini N, Lauthier JJ, Alberti D’Amato AM, et al. Trypanosoma cruzi diversity in the Gran Chaco: mixed infections and differential host distribution of TcV and TcVI. Infect Genet Evol. (2015) 29:53–9. doi: 10.1016/j.meegid.2014.11.001
73. Monje-Rumi MM, Floridia-Yapur N, Zago MP, Ragone PG, Pérez Brandán CM, Nuñez S, et al. Potential association of Trypanosoma cruzi DTUs TcV and TcVI with the digestive form of Chagas disease. Infect Genet Evol. (2020) 84:104329. doi: 10.1016/j.meegid.2020.104329
74. Monteiro WM, Magalhães LK, Santana Filho FS, Borborema M, Silveira H, Barbosa Md. Trypanosoma cruzi TcIII/Z3 genotype as agent of an outbreak of Chagas disease in the Brazilian Western Amazonia. Trop Med Int Health. (2010) 15:1049–51. doi: 10.1111/j.1365-3156.2010.02577.x
75. Garzón EA, Barnabé C, Córdova X, Bowen C, Paredes W, Gómez E, et al. Trypanosoma cruzi isoenzyme variability in Ecuador: first observation of zymodeme III genotypes in chronic chagasic patients. Trans R Soc Trop Med Hyg. (2002) 96:378–82. doi: 10.1016/s0035-9203(02)90367-6
76. Messenger LA, Ramirez JD, Llewellyn MS, Guhl F, Miles MA. Importation of hybrid human-associated trypanosoma cruzi strains of Southern South American origin, Colombia. Emerg Infect Dis. (2016) 22:1452–5. doi: 10.3201/eid2208.150786
77. Brener Z. Comparative studies of different strains of Trypanosoma cruzi. Ann Trop Med Parasitol. (1965) 59:19–26. doi: 10.1080/00034983.1965
78. Brener Z. The behavior of slender and stout forms of Trypanosoma cruzi in the blood-stream of normal and immune mice. Ann Trop Med Parasitol. (1969) 63:215–20. doi: 10.1080/00034983.1969.11686622
79. Tibayrenc M. Modelling the transmission of Trypanosoma cruzi: the need for an integrated genetic epidemiological and population genomics approach. Adv Exp Med Biol. (2010) 673:200–11. doi: 10.1007/978-1-4419-6064-1_14
80. Williams-Blangero S, VandeBerg JL, Blangero J, Corrêa-Oliveira R. Genetic epidemiology of Chagas disease. Adv Parasitol. (2011) 75:147–67. doi: 10.1016/B978-0-12-385863-4.00007-1
81. Silveira-Lemos D, Alessio GD, Batista MA, de Azevedo PO, Reis-Cunha JL, Mendes TAO, et al. Phenotypic, functional and serological aspects of genotypic-specific immune response of experimental T. cruzi infection. Acta Trop. (2021) 222:106021. doi: 10.1016/j.actatropica.2021.106021
82. Nielebock MAP, Moreira OC, Xavier SCDC, Miranda LFC, Lima ACB, Pereira TOJS, et al. Association between Trypanosoma cruzi DTU TcII and chronic Chagas disease clinical presentation and outcome in an urban cohort in Brazil. PloS One. (2020) 15:e0243008. doi: 10.1371/journal.pone.0243008
83. Garcia MN, Burroughs H, Gorchakov R, Gunter SM, Dumonteil E, Murray KO, et al. Molecular identification and genotyping of Trypanosoma cruzi DNA in autochthonous Chagas disease patients from Texas, USA. Infect Genet Evol. (2017) 49:151–6. doi: 10.1016/j.meegid.2017.01.016
84. Toledo MJ, de Lana M, Carneiro CM, Bahia MT, MaChado-Coelho GL, Veloso VM, et al. Impact of Trypanosoma cruzi clonal evolution on its biological properties in mice. Exp Parasitol. (2002) 100:161–72. doi: 10.1016/s0014-4894(02)00003-6
85. Toledo MJ, Bahia MT, Carneiro CM, Martins-Filho OA, Tibayrenc M, Barnabé C, et al. Chemotherapy with benznidazole and itraconazole for mice infected with different Trypanosoma cruzi clonal genotypes. Antimicrob Agents Chemother. (2003) 47:223–30. doi: 10.1128/AAC.47.1.223-230.2003
86. Santos DM, Martins TA, Caldas IS, Diniz LF, MaChado-Coelho GL, Carneiro CM, et al. Benznidazole alters the pattern of Cyclophosphamide-induced reactivation in experimental Trypanosoma cruzi-dependent lineage infection. Acta Trop. (2010) 113:134–8. doi: 10.1016/j.actatropica.2009.10.007
87. Burleigh BA. Probing Trypanosoma cruzi biology with DNA microarrays. Parasitology. (2004) 1:S3–10. doi: 10.1017/S0031182004006559
88. dos Santos DM, Talvani A, Guedes PM, MaChado-Coelho GL, de Lana M, Bahia MT. Trypanosoma cruzi: Genetic diversity influences the profile of immunoglobulins during experimental infection. Exp Parasitol. (2009) 121:8–14. doi: 10.1016/j.exppara.2008.09.012
89. Rodrigues CM, Valadares HM, Francisco AF, Arantes JM, Campos CF, Teixeira-Carvalho A, et al. Coinfection with different Trypanosoma cruzi strains interferes with the host immune response to infection. PloS Negl Trop Dis. (2010) 4:e846. doi: 10.1371/journal.pntd.0000846
90. Meza SK, Kaneshima EN, Silva Sde O, Gabriel M, de Araújo SM, Gomes ML, et al. Comparative pathogenicity in Swiss mice of Trypanosoma cruzi IV from northern Brazil and Trypanosoma cruzi II from southern Brazil. Exp Parasitol. (2014) 146:34–42. doi: 10.1016/j.exppara.2014.08.014
91. Duz AL, Vieira PM, Roatt BM, Aguiar-Soares RD, Cardoso JM, Oliveira FC, et al. The TcI and TcII Trypanosoma cruzi experimental infections induce distinct immune responses and cardiac fibrosis in dogs. Mem Inst Oswaldo Cruz. (2014) 109:1005–13. doi: 10.1590/0074-02760140208
92. Reis MaChado J, Silva MV, Borges DC, da Silva CA, Ramirez LE, dos Reis MA, et al. Immunopathological aspects of experimental Trypanosoma cruzi reinfections. BioMed Res Int. (2014) 2014:648715. doi: 10.1155/2014/648715
93. Magalhães LMD, Passos LSA, Chiari E, Galvão LMC, Koh CC, Rodrigues-Alves ML, et al. Co-infection with distinct Trypanosoma cruzi strains induces an activated immune response in human monocytes. Parasite Immunol. (2019) 41:e12668. doi: 10.1111/pim.12668
94. Martins HR, Toledo MJ, Veloso VM, Carneiro CM, MaChado-Coelho GL, Tafuri WL, et al. Trypanosoma cruzi: Impact of dual-clone infections on parasite biological properties in BALB/c mice. Exp Parasitol. (2006) 112:237–46. doi: 10.1016/j.exppara.2005.11.006
95. Martins HR, Silva RM, Valadares HM, Toledo MJ, Veloso VM, Vitelli-Avelar DM, et al. Impact of dual infections on chemotherapeutic efficacy in BALB/c mice infected with major genotypes of Trypanosoma cruzi. Antimicrob Agents Chemother. (2007) 51:3282–9. doi: 10.1128/AAC.01590-06
96. Martins HR, Figueiredo LM, Valamiel-Silva JC, Carneiro CM, MaChado-Coelho GL, Vitelli-Avelar DM, et al. Persistence of PCR-positive tissue in benznidazole-treated mice with negative blood parasitological and serological tests in dual infections with Trypanosoma cruzi stocks from different genotypes. J Antimicrob Chemother. (2008) 61:1319–27. doi: 10.1093/jac/dkn092
97. Andrade SG, Magalhães JB. Biodemes and zymodemes of Trypanosoma cruzi strains: correlations with clinical data and experimental pathology. Rev Soc Bras Med Trop. (1997) 30:27–35. doi: 10.1590/s0037-86821997000100006
98. Laurent JP, Barnabe C, Quesney V, Noel S, Tibayrenc M. Impact of clonal evolution on the biological diversity of Trypanosoma cruzi. Parasitology. (1997) 114:213–8. doi: 10.1017/s0031182096008414
99. Revollo S, Oury B, Laurent JP, Barnabé C, Quesney V, Carrière V, et al. Trypanosoma cruzi: impact of clonal evolution of the parasite on its biological and medical properties. Exp Parasitol. (1998) 89:30–9. doi: 10.1006/expr.1998.4216
100. Oliveira MT, Branquinho RT, Alessio GD, Mello CGC, Nogueira-de-Paiva NC, Carneiro CM, et al. TcI, TcII and TcVI Trypanosoma cruzi samples from Chagas disease patients with distinct clinical forms and critical analysis of in vitro and in vivo behavior, response to treatment and infection evolution in murine model. Acta Trop. (2017) 167:108–20. doi: 10.1016/j.actatropica.2016.11.033
101. Carneiro M, Romanha AJ, Chiari E. Biological characterization of Trypanosoma cruzi strains from different zymodemes and schizodemes. Mem Inst Oswaldo Cruz. (1991) 86:387–93. doi: 10.1590/s0074-02761991000400002
102. Magalhães JB, Andrade SG, Sherlock I. Trypanosoma cruzi strains: behavior after passage into authoctonous or foreign species of traitomine (biological and biochemical patterns). Rev Inst Med Trop Sao Paulo. (1996) 38:23–8. doi: 10.1590/s0036-46651996000100005
103. de Lana M, da Silveira Pinto A, Barnabé C, Quesney V, Noël S, Tibayrenc M. Trypanosoma cruzi: compared vectorial transmissibility of three major clonal genotypes by Triatoma infestans. Exp Parasitol. (1998) 90:20–5. doi: 10.1006/expr.1998.4304
104. Pinto AS, de Lana M, Bastrenta B, Barnabé C, Quesney V, Noël S, et al. Compared vectorial transmissibility of pure and mixed clonal genotypes of Trypanosoma cruzi in Triatoma infestans. Parasitol Res. (1998) 84:348–53. doi: 10.1007/s004360050409
105. da Silveira Pinto A, de Lana M, Britto C, Bastrenta B, Tibayrenc M. Experimental Trypanosoma cruzi biclonal infection in Triatoma infestans: detection of distinct clonal genotypes using kinetoplast DNA probes. Int J Parasitol. (2000) 30:843–8. doi: 10.1016/s0020-7519(00)00058-8
106. Sandoval-Rodríguez A, Rojo G, López A, Ortiz S, Saavedra M, Botto-Mahan C, et al. Comparing vector competence of Mepraia gajardoi and Triatoma infestans by genotyping Trypanosoma cruzi discrete typing units present in naturally infected Octodon degus. Acta Trop. (2019) 190:119–22. doi: 10.1016/j.actatropica.2018.11.007
107. Rimoldi A, Tomé Alves R, Ambrósio DL, Fernandes MZ, Martinez I, De Araújo RF, et al. Morphological, biological and molecular characterization of three strains of Trypanosoma cruzi Chagas, 1909 (Kinetoplastida, Trypanosomatidae) isolated from Triatoma sordida (Stal) 1859 (Hemiptera, Reduviidae) and a domestic cat. Parasitology. (2012) 139:37–44. doi: 10.1017/S0031182011001697
108. Nogueira-Paiva NC, Vieira PM, Oliveri LM, Fonseca Kda S, Pound-Lana G, de Oliveira MT, et al. Host-Parasite Interactions in Chagas Disease: Genetically Unidentical Isolates of a Single Trypanosoma cruzi Strain Identified In Vitro via LSSP-PCR. PloS One. (2015) 10:e0137788. doi: 10.1371/journal.pone.0137788
109. Mello CB, Azambuja P, Garcia ES, Ratcliffe NA. Differential in vitro and in vivo behavior of three strains of Trypanosoma cruzi in the gut and hemolymph of Rhodnius prolixus. Exp Parasitol. (1996) 82:112–21. doi: 10.1006/expr.1996.0015
110. Vallejo GA, Guhl F, Schaub GA. Triatominae-Trypanosoma cruzi/T. rangeli: Vector-parasite interactions. Acta Trop. (2009) 110:137–47. doi: 10.1016/j.actatropica.2008.10.001
111. de Abreu AP, Lucas da Silva HF, Sarto MPM, Iunklaus GF, Trovo JV, de Souza Fernandes N, et al. Infection susceptibility and vector competence of Rhodnius robustus Larrousse, 1927 and R. pictipes Stal, 1872 (Hemiptera, Reduviidae, Triatominae) for strains of Trypanosoma cruzi (Chagas, 1909) (Kinetoplastida, Trypanosomatidae) I, II and IV. Parasit Vectors. (2022) 15:239. doi: 10.1186/s13071-022-05350-3
112. Andrade SG. Morphological and behavioural characterization of Trypanosoma cruzi strains. Rev Soc Bras Med Trop. (1985) 18:39–46.
113. Lana M, da Silviera Pinto A, Bastrenta B, Barnabé C, Noël S, Tibayrenc M. Trypanosoma cruzi: infectivity of clonal genotype infections in acute and chronic phases in mice. Exp Parasitol. (2000) 96:61–6. doi: 10.1006/expr.2000.4552
114. Andrade SG, Campos RF, Steindel M, Guerreiro ML, Magalhães JB, Almeida MC, et al. Biological, biochemical and molecular features of Trypanosoma cruzi strains isolated from patients infected through oral transmission during a 2005 outbreak in the state of Santa Catarina, Brazil: its correspondence with the new T. cruzi Taxonomy Consensus (2009). Mem Inst Oswaldo Cruz. (2011) 106:948–56. doi: 10.1590/s0074-02762011000800009
115. Ragone PG, Pérez Brandán C, Padilla AM, Monje Rumi M, Lauthier JJ, Alberti D’Amato AM, et al. Biological behavior of different Trypanosoma cruzi isolates circulating in an endemic area for Chagas disease in the Gran Chaco region of Argentina. Acta Trop. (2012) 123:196–201. doi: 10.1016/j.actatropica.2012.05.003
116. Dias GB, Gruendling AP, Araújo SM, Gomes ML, Toledo MJ. Evolution of infection in mice inoculated by the oral route with different developmental forms of Trypanosoma cruzi I and II. Exp Parasitol. (2013) 135:511–7. doi: 10.1016/j.exppara.2013.08.013
117. Monteiro WM, Margioto Teston AP, Gruendling AP, dos Reis D, Gomes ML, de Araújo SM, et al. Trypanosoma cruzi I and IV stocks from Brazilian Amazon are divergent in terms of biological and medical properties in mice. PloS Negl Trop Dis. (2013) 7:e2069. doi: 10.1371/journal.pntd.0002069
118. Teston AP, Monteiro WM, Reis D, Bossolani GD, Gomes ML, de Araújo SM, et al. In vivo susceptibility to benznidazole of Trypanosoma cruzi strains from the western Brazilian Amazon. Trop Med Int Health. (2013) 18:85–95. doi: 10.1111/tmi.12014
119. Magalhães LM, Viana A, Chiari E, Galvão LM, Gollob KJ, Dutra WO. Differential activation of human monocytes and lymphocytes by distinct strains of trypanosoma cruzi. PloS Negl Trop Dis. (2015) 9:e0003816. doi: 10.1371/journal.pntd.0003816
120. Ragone PG, Pérez Brandán C, Monje Rumi M, Tomasini N, Lauthier JJ, Cimino RO, et al. Experimental evidence of biological interactions among different isolates of Trypanosoma cruzi from the Chaco Region. PloS One. (2015) 10:e0119866. doi: 10.1371/journal.pone.0119866
121. Sales-Campos H, Kappel HB, Andrade CP, Lima TP, de Castilho A, Giraldo LE, et al. Trypanosoma cruzi DTU TcII presents higher blood parasitism than DTU TcI in an experimental model of mixed infection. Acta Parasitol. (2015) 60:435–41. doi: 10.1515/ap-2015-0060
122. Lisboa CV, Pinho AP, Monteiro RV, Jansen AM. Trypanosoma cruzi (kinetoplastida Trypanosomatidae): biological heterogeneity in the isolates derived from wild hosts. Exp Parasitol. (2007) 116:150–5. doi: 10.1016/j.exppara.2006.12.005
123. Cura CI, Mejía-Jaramillo AM, Duffy T, Burgos JM, Rodriguero M, Cardinal MV, et al. Trypanosoma cruzi I genotypes in different geographical regions and transmission cycles based on a microsatellite motif of the intergenic spacer of spliced-leader genes. Int J Parasitol. (2010) 40:1599–607. doi: 10.1016/j.ijpara.2010.06.006
124. Andrade LO, MaChado CR, Chiari E, Pena SD, Macedo AM. Differential tissue distribution of diverse clones of Trypanosoma cruzi in infected mice. Mol Biochem Parasitol. (1999) 100:163–72. doi: 10.1016/s0166-6851(99)90035-x
125. Vago AR, Andrade LO, Leite AA, d’Avila Reis D, Macedo AM, Adad SJ, et al. Genetic characterization of Trypanosoma cruzi directly from tissues of patients with chronic Chagas disease: differential distribution of genetic types into diverse organs. Am J Pathol. (2000) 156:1805–9. doi: 10.1016/s0002-9440(10)65052-3
126. Mantilla JC, Zafra GA, Macedo AM, González CI. Mixed infection of Trypanosoma cruzi I and II in a Colombian cardiomyopathic patient. Hum Pathol. (2010) 41:610–3. doi: 10.1016/j.humpath.2009.11.005
127. Sales-Campos H, Kappel HB, Andrade CP, Lima TP, Mattos ME Jr, de Castilho A, et al. A DTU-dependent blood parasitism and a DTU-independent tissue parasitism during mixed infection of Trypanosoma cruzi in immunosuppressed mice. Parasitol Res. (2014) 113:375–85. doi: 10.1007/s00436-013-3665-z
128. Perez CJ, Thompson RCA, Keatley SK, Walsh AL, Lymbery AJ. The effect of reinfection and mixed Trypanosoma cruzi infections on disease progression in mice. Acta Trop. (2018) 178:107–14. doi: 10.1016/j.actatropica.2017.11.002
129. Franco DJ, Vago AR, Chiari E, Meira FC, Galvão LM, MaChado CR. Trypanosoma cruzi: mixture of two populations can modify virulence and tissue tropism in rat. Exp Parasitol. (2003) 104:54–61. doi: 10.1016/s0014-4894(03)00119-x
130. Andrade SG, Campos RF, Sobral KS, Magalhães JB, Guedes RS, Guerreiro ML. Reinfections with strains of Trypanosoma cruzi, of different biodemes as a factor of aggravation of myocarditis and myositis in mice. Rev Soc Bras Med Trop. (2006) 39:1–8. doi: 10.1590/s0037-86822006000100001
131. Dutra WO, Menezes CA, Villani FN, da Costa GC, da Silveira AB, Reis Dd, et al. Cellular and genetic mechanisms involved in the generation of protective and pathogenic immune responses in human Chagas disease. Mem Inst Oswaldo Cruz. (2009) 104:208–18. doi: 10.1590/s0074-02762009000900027
132. Ferreira BL, Ferreira ÉR, de Brito MV, Salu BR, Oliva MLV, Mortara RA, et al. BALB/c and C57BL/6 mice cytokine responses to trypanosoma cruzi infection are independent of parasite strain infectivity. Front Microbiol. (2018) 9:553. doi: 10.3389/fmicb.2018.00553
133. Gonçalves da Costa SC, Calabrese KS, Zaverucha do Valle T, Lagrange PH. Trypanosoma cruzi: infection patterns in intact and athymic mice of susceptible and resistant genotypes. Histol Histopathol. (2002) 17:837–44. doi: 10.14670/HH-17.837
134. Sanoja C, Carbajosa S, Fresno M, Gironès N. Analysis of the dynamics of infiltrating CD4(+) T cell subsets in the heart during experimental Trypanosoma cruzi infection. PloS One. (2013) 8:e65820. doi: 10.1371/journal.pone.0065820
135. Domingues CS, Cardoso FO, Hardoim DJ, Pelajo-MaChado M, Bertho AL, Calabrese KDS. Host genetics background influence in the intragastric trypanosoma cruzi infection. Front Immunol. (2020) 11:566476. doi: 10.3389/fimmu.2020.566476
136. Frade AF, Pissetti CW, Ianni BM, Saba B, Lin-Wang HT, Nogueira LG, et al. Genetic susceptibility to Chagas disease cardiomyopathy: involvement of several genes of the innate immunity and chemokine-dependent migration pathways. BMC Infect Dis. (2013) 13:587. doi: 10.1186/1471-2334-13-587
137. Luz PR, Miyazaki MI, Chiminacio Neto N, Padeski MC, Barros AC, Boldt AB, et al. Genetically determined MBL deficiency is associated with protection against chronic cardiomyopathy in chagas disease. PloS Negl Trop Dis. (2016) 10:e0004257. doi: 10.1371/journal.pntd.0004257
138. de Souza W, de Carvalho TM, Barrias ES. Review on trypanosoma cruzi: host cell interaction. Int J Cell Biol. (2010) 2010:295394. doi: 10.1155/2010/295394
139. Maeda FY, Cortez C, Yoshida N. Cell signaling during Trypanosoma cruzi invasion. Front Immunol. (2012) 3:361. doi: 10.3389/fimmu.2012.00361
140. Villalta F, Madison MN, Kleshchenko YY, Nde PN, Lima MF. Molecular analysis of early host cell infection by Trypanosoma cruzi. Front Biosci. (2008) 13:3714–34. doi: 10.2741/2961
141. Nagajyothi F, Desruisseaux MS, MaChado FS, Upadhya R, Zhao D, Schwartz GJ, et al. Response of adipose tissue to early infection with Trypanosoma cruzi (Brazil strain). J Infect Dis. (2012) 205:830–40. doi: 10.1093/infdis/jir840
142. Nakayasu ES, Sobreira TJ, Torres R Jr, Ganiko L, Oliveira PS, Marques AF, et al. Improved proteomic approach for the discovery of potential vaccine targets in Trypanosoma cruzi. J Proteome Res. (2012) 11:237–46. doi: 10.1021/pr200806s
143. Andrews NW, Colli W. Adhesion and interiorization of Trypanosoma cruzi in mammalian cells. J Protozool. (1982) 29:264–9. doi: 10.1111/j.1550-7408.1982.tb04024.x
144. Bayer-Santos E, Aguilar-Bonavides C, Rodrigues SP, Cordero EM, Marques AF, Varela-Ramirez A, et al. Proteomic analysis of Trypanosoma cruzi secretome: characterization of two populations of extracellular vesicles and soluble proteins. J Proteome Res. (2013) 12:883–97. doi: 10.1021/pr300947g
145. De Pablos LM, Osuna A. Multigene families in Trypanosoma cruzi and their role in infectivity. Infect Immun. (2012) 80:2258–64. doi: 10.1128/IAI.06225-11
146. Marques AF, Nakayasu ES, Almeida IC. Purification of extracellular and intracellular amastigotes of Trypanosoma cruzi from mammalian host-infected cells. Protocol Exchange. (2011). doi: 10.1038/protex.2011.265
147. Singh H, Raghava GP. ProPred1: prediction of promiscuous MHC Class-I binding sites. Bioinformatics. (2003) 19:1009–14. doi: 10.1093/bioinformatics/btg108
148. Serna C, Lara JA, Rodrigues SP, Marques AF, Almeida IC, Maldonado RA. A synthetic peptide from Trypanosoma cruzi mucin-like associated surface protein as candidate for a vaccine against Chagas disease. Vaccine. (2014) 32:3525–32. doi: 10.1016/j.vaccine.2014.04.026
149. Nogueira N. Host and parasite factors affecting the invasion of mononuclear phagocytes by Trypanosoma cruzi. Ciba Found Symp. (1983) 99:52–73. doi: 10.1002/9780470720806.ch4
150. Yoshida N. Molecular mechanisms of Trypanosoma cruzi infection by oral route. Mem Inst Oswaldo Cruz. (2009) 104:101–7. doi: 10.1590/s0074-02762009000900015
151. Lima MF, Villalta F. Host-cell attachment by Trypanosoma cruzi: identification of an adhesion molecule. Biochem Biophys Res Commun. (1988) 155:256–62. doi: 10.1016/s0006-291x(88)81077-5
152. Gazzinelli RT, Ropert C, Campos MA. Role of the Toll/interleukin-1 receptor signaling pathway in host resistance and pathogenesis during infection with protozoan parasites. Immunol Rev. (2004) 201:9–25. doi: 10.1111/j.0105-2896.2004.00174.x
153. Kawasaki T, Kawai T. Toll-like receptor signaling pathways. Front Immunol. (2014) 5:461. doi: 10.3389/fimmu.2014.00461
154. Castillo C, Muñoz L, Carrillo I, Liempi A, Gallardo C, Galanti N, et al. Ex vivo infection of human placental chorionic villi explants with Trypanosoma cruzi and Toxoplasma gondii induces different Toll-like receptor expression and cytokine/chemokine profiles. Am J Reprod Immunol. (2017) 78(1). doi: 10.1111/aji.12660
155. Blasius AL, Beutler B. Intracellular toll-like receptors. Immunity. (2010) 32:305–15. doi: 10.1016/j.immuni.2010.03.012
156. Akira S, Uematsu S, Takeuchi O. Pathogen recognition and innate immunity. Cell. (2006) 124:783–801. doi: 10.1016/j.cell.2006.02.015
157. Schmitz V, Svensjö E, Serra RR, Teixeira MM, Scharfstein J. Proteolytic generation of kinins in tissues infected by Trypanosoma cruzi depends on CXC chemokine secretion by macrophages activated via Toll-like 2 receptors. J Leukoc Biol. (2009) 85:1005–14. doi: 10.1189/jlb.1108693
158. Gazzinelli RT, Hieny S, Wynn TA, Wolf S, Sher A. Interleukin 12 is required for the T-lymphocyte-independent induction of interferon gamma by an intracellular parasite and induces resistance in T-cell-deficient hosts. Proc Natl Acad Sci U.S.A. (1993) 90:6115–9. doi: 10.1073/pnas.90.13.6115
159. Kayama H, Takeda K. The innate immune response to Trypanosoma cruzi infection. Microbes Infect. (2010) 12:511–7. doi: 10.1016/j.micinf.2010.03.005
160. Muñoz-Fernández MA, Fernández MA, Fresno M. Synergism between tumor necrosis factor-alpha and interferon-gamma on macrophage activation for the killing of intracellular Trypanosoma cruzi through a nitric oxide-dependent mechanism. Eur J Immunol. (1992) 22:301–7. doi: 10.1002/eji.1830220203
161. Vespa GN, Cunha FQ, Silva JS. Nitric oxide is involved in control of Trypanosoma cruzi-induced parasitemia and directly kills the parasite in vitro. Infect Immun. (1994) 62:5177–82. doi: 10.1128/iai.62.11.5177-5182.1994
162. Alvarez MN, Peluffo G, Piacenza L, Radi R. Intraphagosomal peroxynitrite as a macrophage-derived cytotoxin against internalized Trypanosoma cruzi: consequences for oxidative killing and role of microbial peroxiredoxins in infectivity. J Biol Chem. (2011) 286:6627–40. doi: 10.1074/jbc.M110.167247
163. Parodi C, Padilla AM, Basombrío MA. Protective immunity against Trypanosoma cruzi. Mem Inst Oswaldo Cruz. (2009) 104:288–94. doi: 10.1590/s0074-02762009000900038
164. Padilla AM, Bustamante JM, Tarleton RL. CD8+ T cells in Trypanosoma cruzi infection. Curr Opin Immunol. (2009) 21:385–90. doi: 10.1016/j.coi.2009.07.006
165. Ricklin D, Hajishengallis G, Yang K, Lambris JD. Complement: a key system for immune surveillance and homeostasis. Nat Immunol. (2010) 11:785–97. doi: 10.1038/ni.1923
166. Cardoso MS, Reis-Cunha JL, Bartholomeu DC. Evasion of the Immune Response by Trypanosoma cruzi during Acute Infection. Front Immunol. (2016) 6:659. doi: 10.3389/fimmu.2015.00659
167. Lidani KCF, Bavia L, Ambrosio AR, de Messias-Reason IJ. The complement system: A prey of trypanosoma cruzi. Front Microbiol. (2017) 8:607. doi: 10.3389/fmicb.2017.00607
168. Ferreira V, Valck C, Sánchez G, Gingras A, Tzima S, Molina MC, et al. The classical activation pathway of the human complement system is specifically inhibited by calreticulin from Trypanosoma cruzi. J Immunol. (2004) 172:3042–50. doi: 10.4049/jimmunol.172.5.3042
169. Souto-Padrón T, Labriola CA, de Souza W. Immunocytochemical localisation of calreticulin in Trypanosoma cruzi. Histochem Cell Biol. (2004) 122:563–9. doi: 10.1007/s00418-004-0724-7
170. González A, Valck C, Sánchez G, Härtel S, Mansilla J, Ramírez G, et al. Trypanosoma cruzi calreticulin topographical variations in parasites infecting murine macrophages. Am J Trop Med Hyg. (2015) 92:887–97. doi: 10.4269/ajtmh.14-0497
171. Ramírez G, Valck C, Molina MC, Ribeiro CH, López N, Sánchez G, et al. Trypanosoma cruzi calreticulin: a novel virulence factor that binds complement C1 on the parasite surface and promotes infectivity. Immunobiology. (2011) 216:265–73. doi: 10.1016/j.imbio.2010.04.001
172. Sosoniuk E, Vallejos G, Kenawy H, Gaboriaud C, Thielens N, Fujita T, et al. Trypanosoma cruzi calreticulin inhibits the complement lectin pathway activation by direct interaction with L-Ficolin. Mol Immunol. (2014) 60:80–5. doi: 10.1016/j.molimm.2014.03.014
173. Norris KA, Bradt B, Cooper NR, So M. Characterization of a Trypanosoma cruzi C3 binding protein with functional and genetic similarities to the human complement regulatory protein, decay-accelerating factor. J Immunol. (1991) 147:2240–7.
174. Inal JM, Schifferli JA. Complement C2 receptor inhibitor trispanning and the beta-chain of C4 share a binding site for complement C2. J Immunol. (2002) 168:5213–21. doi: 10.4049/jimmunol.168.10.5213
175. Inal JM. Complement C2 receptor inhibitor trispanning: from man to schistosome. Springer Semin Immunopathol. (2005) 27:320–31. doi: 10.1007/s00281-005-0009-9
176. Cestari I, Ansa-Addo E, Deolindo P, Inal JM, Ramirez MI. Trypanosoma cruzi immune evasion mediated by host cell-derived microvesicles. J Immunol. (2012) 188:1942–52. doi: 10.4049/jimmunol.1102053
177. Wyllie MP, Ramirez MI. Microvesicles released during the interaction between Trypanosoma cruzi TcI and TcII strains and host blood cells inhibit complement system and increase the infectivity of metacyclic forms of host cells in a strain-independent process. Pathog Dis. (2017) 75(7). doi: 10.1093/femspd/ftx077
178. Fischer E, Ouaissi MA, Velge P, Cornette J, Kazatchkine MD. gp 58/68, a parasite component that contributes to the escape of the trypomastigote form of T. cruzi from damage by the human alternative complement pathway. Immunology. (1988) 65:299–303.
179. Ouaissi MA, Afchain D, Capron A, Grimaud JA. Fibronectin receptors on Trypanosoma cruzi trypomastigotes and their biological function. Nature. (1984) 308:380–2. doi: 10.1038/308380a0
180. Joiner KA, daSilva WD, Rimoldi MT, Hammer CH, Sher A, Kipnis TL. Biochemical characterization of a factor produced by trypomastigotes of Trypanosoma cruzi that accelerates the decay of complement C3 convertases. J Biol Chem. (1988) 263:11327–35.
181. Tambourgi DV, Kipnis TL, da Silva WD, Joiner KA, Sher A, Heath S, et al. A partial cDNA clone of trypomastigote decay-accelerating factor (T-DAF), a developmentally regulated complement inhibitor of Trypanosoma cruzi, has genetic and functional similarities to the human complement inhibitor DAF. Infect Immun. (1993) 61:3656–63. doi: 10.1128/iai.61.9.3656-3663.1993
182. Camussi G, Deregibus MC, Bruno S, Cantaluppi V, Biancone L. Exosomes/microvesicles as a mechanism of cell-to-cell communication. Kidney Int. (2010) 78:838–48. doi: 10.1038/ki.2010.278
183. Stahl PD, Raposo G. Extracellular vesicles: exosomes and microvesicles, integrators of homeostasis. Physiol (Bethesda). (2019) 34:169–77. doi: 10.1152/physiol.00045.2018
184. Quijano-Hernandez I, Dumonteil E. Advances and challenges towards a vaccine against Chagas disease. Hum Vaccin. (2011) 7:1184–91. doi: 10.4161/hv.7.11.17016
185. Brener Z, Gazzinelli RT. Immunological control of Trypanosoma cruzi infection and pathogenesis of Chagas’ disease. Int Arch Allergy Immunol. (1997) 114:103–10. doi: 10.1159/000237653
186. Vitelli-Avelar DM, Sathler-Avelar R, Dias JC, Pascoal VP, Teixeira-Carvalho A, Lage PS, et al. Chagasic patients with indeterminate clinical form of the disease have high frequencies of circulating CD3+CD16-CD56+ natural killer T cells and CD4+CD25High regulatory T lymphocytes. Scand J Immunol. (2005) 62:297–308. doi: 10.1111/j.1365-3083.2005.01668.x
187. Sathler-Avelar R, Vitelli-Avelar DM, Teixeira-Carvalho A, Martins-Filho OA. Innate immunity and regulatory T-cells in human Chagas disease: what must be understood? Mem Inst Oswaldo Cruz. (2009) 104:246–51. doi: 10.1590/s0074-02762009000900031
188. Gomes JA, Bahia-Oliveira LM, Rocha MO, Martins-Filho OA, Gazzinelli G, Correa-Oliveira R. Evidence that development of severe cardiomyopathy in human Chagas’ disease is due to a Th1-specific immune response. Infect Immun. (2003) 71:1185–93. doi: 10.1128/IAI.71.3.1185-1193.2003
189. Liu G, Zhao Y. Toll-like receptors and immune regulation: their direct and indirect modulation on regulatory CD4+ CD25+ T cells. Immunology. (2007) 122:149–56. doi: 10.1111/j.1365-2567.2007.02651.x
190. Dutra WO, Menezes CA, Magalhães LM, Gollob KJ. Immunoregulatory networks in human Chagas disease. Parasite Immunol. (2014) 36:377–87. doi: 10.1111/pim.12107
191. Araujo FF, Gomes JA, Rocha MO, Williams-Blangero S, Pinheiro VM, Morato MJ, et al. Potential rolefaz of CD4+CD25HIGH regulatory T cells in morbidity in Chagas disease. Front Biosci. (2007) 12:2797–806. doi: 10.2741/2273
192. Costa GC, da Costa Rocha MO, Moreira PR, Menezes CA, Silva MR, Gollob KJ, et al. Functional IL-10 gene polymorphism is associated with Chagas disease cardiomyopathy. J Infect Dis. (2009) 199:451–4. doi: 10.1086/596061
193. Reis DD, Jones EM, Tostes S Jr, Lopes ER, Gazzinelli G, Colley DG, et al. Characterization of inflammatory infiltrates in chronic chagasic myocardial lesions: presence of tumor necrosis factor-alpha+ cells and dominance of granzyme A+, CD8+ lymphocytes. Am J Trop Med Hyg. (1993) 48:637–44. doi: 10.4269/ajtmh.1993.48.637
194. Souza PE, Rocha MO, Rocha-Vieira E, Menezes CA, Chaves AC, Gollob KJ, et al. Monocytes from patients with indeterminate and cardiac forms of Chagas’ disease display distinct phenotypic and functional characteristics associated with morbidity. Infect Immun. (2004) 72:5283–91. doi: 10.1128/IAI.72.9.5283-5291.2004
195. Vitelli-Avelar DM, Sathler-Avelar R, Teixeira-Carvalho A, Pinto Dias JC, Gontijo ED, Faria AM, et al. Strategy to assess the overall cytokine profile of circulating leukocytes and its association with distinct clinical forms of human Chagas disease. Scand J Immunol. (2008) 68:516–25. doi: 10.1111/j.1365-3083.2008.02167.x
196. Andrade LO, Andrews NW. The Trypanosoma cruzi-host-cell interplay: location, invasion, retention. Nat Rev Microbiol. (2005) 3:819–23. doi: 10.1038/nrmicro1249
197. Coura JR. Chagas disease: what is known and what is needed–a background article. Mem Inst Oswaldo Cruz. (2007) 102:113–22. doi: 10.1590/s0074-02762007000900018
198. Dias JCP, Coura JR. Clı́nica e Terapęutica da Doença de Chagas: uma Abordagem Prática para o Clı́nico Geral, Fundaçăo Oswaldo Cruz, Rio de Janeiro. Epidemiologia. (1997). p. 486.
199. Rassi A Jr, Rassi A, Marcondes de Rezende J. American trypanosomiasis (Chagas disease). Infect Dis Clin North Am. (2012) 26:275–91. doi: 10.1016/j.idc.2012.03.002
200. Bonney KM, Luthringer DJ, Kim SA, Garg NJ, Engman DM. Pathology and pathogenesis of chagas heart disease. Annu Rev Pathol. (2019) 14:421–47. doi: 10.1146/annurev-pathol-020117-043711
201. Rossi MA, Ramos SG, Bestetti RB. Chagas’ heart disease: clinical-pathological correlation. Front Biosci. (2003) 8:e94–109. doi: 10.2741/948
202. Rossi MA. Patterns of myocardial fibrosis in idiopathic cardiomyopathies and chronic Chagasic cardiopathy. Can J Cardiol. (1991) 7:287–94.
203. Rossi MA. Fibrosis and inflammatory cells in human chronic chagasic myocarditis: scanning electron microscopy and immunohistochemical observations. Int J Cardiol. (1998) 66:183–94. doi: 10.1016/s0167-5273(98)00208-3
204. Guedes PM, Veloso VM, Afonso LC, Caliari MV, Carneiro CM, Diniz LF, et al. Development of chronic cardiomyopathy in canine Chagas disease correlates with high IFN-gamma, TNF-alpha, and low IL-10 production during the acute infection phase. Vet Immunol Immunopathol. (2009) 130:43–52. doi: 10.1016/j.vetimm.2009.01.004
205. Diniz Lde F, Caldas IS, Guedes PM, Crepalde G, de Lana M, Carneiro CM, et al. Effects of ravuconazole treatment on parasite load and immune response in dogs experimentally infected with Trypanosoma cruzi. Antimicrob Agents Chemother. (2010) 54:2979–86. doi: 10.1128/AAC.01742-09
206. Caliari MV, do Pilar MaChado R, de Lana M, Caja RA, Carneiro CM, Bahia MT, et al. Quantitative analysis of cardiac lesions in chronic canine chagasic cardiomyopathy. Rev Inst Med Trop Sao Paulo. (2002) 44:273–8. doi: 10.1590/s0036-46652002000500008
207. Ferrer MF, Pascuale CA, Gomez RM, Leguizamón MS. DTU I isolates of Trypanosoma cruzi induce upregulation of Galectin-3 in murine myocarditis and fibrosis. Parasitology. (2014) 141:849–58. doi: 10.1017/S0031182013002254
208. Shrestha D, Bajracharya B, Paula-Costa G, Salles BC, Leite AL, Menezes AP, et al. Expression and production of cardiac angiogenic mediators depend on the Trypanosoma cruzi-genetic population in experimental C57BL/6 mice infection. Microvasc Res. (2017) 110:56–63. doi: 10.1016/j.mvr.2016.12.002
209. Köberle F. Chagas’ disease and Chagas’ syndromes: the pathology of American trypanosomiasis. Adv Parasitol. (1968) 6:63–116. doi: 10.1016/s0065-308x(08)60472-8
210. Kalil J, Cunha-Neto E. Autoimmunity in chagas disease cardiomyopathy: Fulfilling the criteria at last? Parasitol Today. (1996) 12:396–9. doi: 10.1016/0169-4758(96)10058-2
211. Vago AR, Macedo AM, Adad SJ, Reis DD, Corrêa-Oliveira R. PCR detection of Trypanosoma cruzi DNA in oesophageal tissues of patients with chronic digestive Chagas’ disease. Lancet. (1996) 348:891–2. doi: 10.1016/S0140-6736(05)64761-7
212. Tarleton RL, Zhang L, Downs MO. “Autoimmune rejection” of neonatal heart transplants in experimental Chagas disease is a parasite-specific response to infected host tissue. Proc Natl Acad Sci U.S.A. (1997) 94:3932–7. doi: 10.1073/pnas.94.8.3932
213. Zhang L, Tarleton RL. Parasite persistence correlates with disease severity and localization in chronic Chagas’ disease. J Infect Dis. (1999) 180:480–6. doi: 10.1086/314889
214. MaChado FS, Dutra WO, Esper L, Gollob KJ, Teixeira MM, Factor SM, et al. Current understanding of immunity to Trypanosoma cruzi infection and pathogenesis of Chagas disease. Semin Immunopathol. (2012) 34:753–70. doi: 10.1007/s00281-012-0351-7
215. Jones EM, Colley DG, Tostes S, Lopes ER, Vnencak-Jones CL, McCurley TL. Amplification of a Trypanosoma cruzi DNA sequence from inflammatory lesions in human chagasic cardiomyopathy. Am J Trop Med Hyg. (1993) 48:348–57. doi: 10.4269/ajtmh.1993.48.348
216. Williams JT, Dick EJ Jr, VandeBerg JL, Hubbard GB. Natural Chagas disease in four baboons. J Med Primatol. (2009) 38:107–13. doi: 10.1111/j.1600-0684.2008.00308.x
217. Jansen AM, Xavier SC, Roque AL. The multiple and complex and changeable scenarios of the Trypanosoma cruzi transmission cycle in the sylvatic environment. Acta Trop. (2015) 151:1–15. doi: 10.1016/j.actatropica.2015.07.018
218. Russo M, Starobinas N, Marcondes MC, Minoprio P, Honteyberie-Joskowicz M. The influence of T cell subsets on Trypanosoma cruzi multiplication in different organs. Immunol Lett. (1996) 49:163–8. doi: 10.1016/0165-2478(96)02498-4
219. Tarleton RL. Parasite persistence in the aetiology of Chagas disease. Int J Parasitol. (2001) 31:550–4. doi: 10.1016/s0020-7519(01)00158-8
220. Macedo AM, Pena SD. Genetic variability of trypanosoma cruzi: implications for the pathogenesis of chagas disease. Parasitol Today. (1998) 14:119–24. doi: 10.1016/s0169-4758(97)01179-4
221. Macedo AM, MaChado CR, Oliveira RP, Pena SD. Trypanosoma cruzi: genetic structure of populations and relevance of genetic variability to the pathogenesis of chagas disease. Mem Inst Oswaldo Cruz. (2004) 99:1–12. doi: 10.1590/s0074-02762004000100001
222. Melo RC, Brener Z. Tissue tropism of different Trypanosoma cruzi strains. J Parasitol. (1978) 64:475–82.
223. Andrade SG. Influence of Trypanosoma cruzi strain on the pathogenesis of chronic myocardiopathy in mice. Mem Inst Oswaldo Cruz. (1990) 85:17–27. doi: 10.1590/s0074-02761990000100003
224. Postan M, McDaniel JP, Dvorak JA. Trypanosoma cruzi: constancy of clone pathogenicity for inbred mice during long-term in vitro maintenance. Trans R Soc Trop Med Hyg. (1986) 80:659–62. doi: 10.1016/0035-9203(86)90166-5
225. Penin P, De Diego J, Del Rey J, Mayer R, Gamallo C. Acute chagasic cardiopathy produced by a strain of Trypanosoma cruzi (type I) in an experimental model. Ann Parasitol Hum Comp. (1990) 65:208–13. doi: 10.1051/parasite/1990655208
226. Lenzi HL, Castelo-Branco MT, Pelajo-MaChado M, Oliveira DN, Gattass CR. Trypanosoma cruzi: compromise of reproductive system in acute murine infection. Acta Trop. (1998) 71:117–29. doi: 10.1016/s0001-706x(98)00058-8
227. de Diego JA, Palau MT, Gamallo C, Penin P. Relationships between histopathological findings and phylogenetic divergence in Trypanosoma cruzi. Trop Med Int Health. (1998) 3:222–33. doi: 10.1111/j.1365-3156.1998.tb00275.x
228. D’Avila DA, Macedo AM, Valadares HM, Gontijo ED, de Castro AM, MaChado CR, et al. Probing population dynamics of Trypanosoma cruzi during progression of the chronic phase in chagasic patients. J Clin Microbiol. (2009) 47:1718–25. doi: 10.1128/JCM.01658-08
229. Vianna G. Contribuição para o estudo da anatomía patológica da “Moléstia de Carlos Chagas”: esquizotripanose humana ou tireoidite parasitária. Mem Inst Oswaldo Cruz. (1911) 3:276–94. doi: 10.1590/S0074-02761911000200004
230. Postan M, McDaniel JP, Dvorak JA. Comparative studies of the infection of Lewis rats with four Trypanosoma cruzi clones. Trans R Soc Trop Med Hyg. (1987) 81:415–9. doi: 10.1016/0035-9203(87)90155-6
231. Macedo AM, Oliveira RP, Pena SD. Chagas disease: role of parasite genetic variation in pathogenesis. Expert Rev Mol Med. (2002) 4:1–16. doi: 10.1017/S1462399402004118
232. Andrade LO, MaChado CR, Chiari E, Pena SD, Macedo AM. Trypanosoma cruzi: role of host genetic background in the differential tissue distribution of parasite clonal populations. Exp Parasitol. (2002) 100:269–75. doi: 10.1016/s0014-4894(02)00024-3
233. Manoel-Caetano Fda S, Silva AE. Implications of genetic variability of Trypanosoma cruzi for the pathogenesis of Chagas disease. Cad Saude Publica. (2007) 23:2263–74. doi: 10.1590/s0102-311x2007001000002
234. Lo Presti MS, Esteves BH, Moya D, Bazán PC, Strauss M, Báez AL, et al. Circulating Trypanosoma cruzi populations differ from those found in the tissues of the same host during acute experimental infection. Acta Trop. (2014) 133:98–109. doi: 10.1016/j.actatropica.2014.02.010
235. Pena DA, Eger I, Nogueira L, Heck N, Menin Á, Báfica A, et al. Selection of TcII Trypanosoma cruzi population following macrophage infection. J Infect Dis. (2011) 204:478–86. doi: 10.1093/infdis/jir292
236. Strauss M, Velázquez López DA, Moya DM, Bazán PC, Báez AL, Rivarola HW, et al. Differential tissue distribution of Trypanosoma cruzi during acute experimental infection: Further evidence using natural isolates. Mol Biochem Parasitol. (2018) 222:29–33. doi: 10.1016/j.molbiopara.2018.04.007
237. Campbell DA, Westenberger SJ, Sturm NR. The determinants of Chagas disease: connecting parasite and host genetics. Curr Mol Med. (2004) 4:549–62. doi: 10.2174/1566524043360249
238. Sartori AM, Shikanai-Yasuda MA, Amato Neto V, Lopes MH. Follow-up of 18 patients with human immunodeficiency virus infection and chronic Chagas’ disease, with reactivation of Chagas’ disease causing cardiac disease in three patients. Clin Infect Dis. (1998) 26:177–9. doi: 10.1086/516257
239. da Costa SC. Immunocompromised host: from the early events until the impact of acquired immunodeficiency syndrome. Mem Inst Oswaldo Cruz. (2000) 1:141–4. doi: 10.1590/s0074-02762000000700023
240. Gontijo CM, Pacheco RS, Orefice F, Lasmar E, Silva ES, Melo MN. Concurrent cutaneous, visceral and ocular leishmaniasis caused by Leishmania (Viannia) Braziliensis in a kidney transplant patient. Mem Inst Oswaldo Cruz. (2002) 97:751–3. doi: 10.1590/s0074-02762002000500029
241. Kotton CN. Zoonoses in solid-organ and hematopoietic stem cell transplant recipients. Clin Infect Dis. (2007) 44:857–66. doi: 10.1086/511859
242. Diazgranados CA, Saavedra-Trujillo CH, Mantilla M, Valderrama SL, Alquichire C, Franco-Paredes C. Chagasic encephalitis in HIV patients: common presentation of an evolving epidemiological and clinical association. Lancet Infect Dis. (2009) 9:324–30. doi: 10.1016/S1473-3099(09)70088-X
243. Solari A, Saavedra H, Sepúlveda C, Oddó D, Acuña G, Labarca J, et al. Successful treatment of Trypanosoma cruzi encephalitis in a patient with hemophilia and AIDS. Clin Infect Dis. (1993) 16:255–9. doi: 10.1093/clind/16.2.255
244. Cordova E, Boschi A, Ambrosioni J, Cudos C, Corti M. Reactivation of Chagas disease with central nervous system involvement in HIV-infected patients in Argentina, 1992-2007. Int J Infect Dis. (2008) 12:587–92. doi: 10.1016/j.ijid.2007.12.007
245. Lattes R, Lasala MB. Chagas disease in the immunosuppressed patient. Clin Microbiol Infect. (2014) 20:300–9. doi: 10.1111/1469-0691.12585
246. Rosemberg S, Chaves CJ, Higuchi ML, Lopes MB, Castro LH, MaChado LR. Fatal meningoencephalitis caused by reactivation of Trypanosoma cruzi infection in a patient with AIDS. Neurology. (1992) 42:640–2. doi: 10.1212/wnl.42.3.640
247. Nishioka Sde A, Ferreira MS, Rocha A, Burgarelli MK, Silva AM, Duarte MI, et al. Reactivation of Chagas’ disease successfully treated with benznidazole in a patient with acquired immunodeficiency syndrome. Mem Inst Oswaldo Cruz. (1993) 88:493–6. doi: 10.1590/s0074-02761993000300022
248. Rocha A, de Meneses AC, da Silva AM, Ferreira MS, Nishioka SA, Burgarelli MK, et al. Pathology of patients with Chagas’ disease and acquired immunodeficiency syndrome. Am J Trop Med Hyg. (1994) 50:261–8. doi: 10.4269/ajtmh.1994.50.261
249. Benatti RD, Oliveira GH, Bacal F. Heart transplantation for chagas cardiomyopathy. J Heart Lung Transplant. (2017) 36:597–603. doi: 10.1016/j.healun.2017.02.006
250. Vieira JL, Távora FRF, Sobral MGV, Vasconcelos GG, Almeida GPL, Fernandes JR, et al. Chagas cardiomyopathy in latin America review. Curr Cardiol Rep. (2019) 21:8. doi: 10.1007/s11886-019-1095-y
251. Moreira MDCV, Renan Cunha-Melo J. Chagas disease infection reactivation after heart transplant. Trop Med Infect Dis. (2020) 5:106. doi: 10.3390/tropicalmed5030106
252. Zingales B, Macedo AM. Fifteen Years after the Definition of Trypanosoma cruzi DTUs: What Have We Learned? Life (Basel). (2023) 13:2339. doi: 10.3390/life13122339
253. Blanche C, Aleksic I, Takkenberg JJ, Czer LS, Fishbein MC, Trento A. Heart transplantation for Chagas’ cardiomyopathy. Ann Thorac Surg. (1995) 60:1406–8; discussion 1408-9. doi: 10.1016/0003-4975(95)00726-2
254. Kransdorf EP, Czer LS, Luthringer DJ, Patel JK, Montgomery SP, Velleca A, et al. Heart transplantation for Chagas cardiomyopathy in the United States. Am J Transplant. (2013) 13:3262–8. doi: 10.1111/ajt.12507
255. Diez M, Favaloro L, Bertolotti A, Burgos JM, Vigliano C, Lastra MP, et al. Usefulness of PCR strategies for early diagnosis of Chagas’ disease reactivation and treatment follow-up in heart transplantation. Am J Transplant. (2007) 7:1633–40. doi: 10.1111/j.1600-6143.2007.01820.x
256. de Souza MM, Franco M, Almeida DR, Diniz RV, Mortara RA, da Silva S, et al. Comparative histopathology of endomyocardial biopsies in chagasic and non-chagasic heart transplant recipients. J Heart Lung Transplant. (2001) 20:534–43. doi: 10.1016/s1053-2498(00)00320-x
257. Sartori AM, Ibrahim KY, Nunes Westphalen EV, Braz LM, Oliveira OC Jr, Gakiya E, et al. Manifestations of Chagas disease (American trypanosomiasis) in patients with HIV/AIDS. Ann Trop Med Parasitol. (2007) 101:31–50. doi: 10.1179/136485907X154629
258. Sánchez-Valdéz FJ, Padilla A, Wang W, Orr D, Tarleton RL. Spontaneous dormancy protects Trypanosoma cruzi during extended drug exposure. Elife. (2018) 7:e34039. doi: 10.7554/eLife.34039
259. Pacheco RS, Ferreira MS, MaChado MI, Brito CM, Pires MQ, Da-Cruz AM, et al. Chagas’ disease and HIV co-infection: genotypic characterization of the Trypanosoma cruzi strain. Mem Inst Oswaldo Cruz. (1998) 93:165–9. doi: 10.1590/s0074-02761998000200005
260. Lages-Silva E, Ramirez LE, Silva-Vergara ML, Chiari E. Chagasic meningoencephalitis in a patient with acquired immunodeficiency syndrome: diagnosis, follow-up, and genetic characterization of Trypanosoma cruzi. Clin Infect Dis. (2002) 34:118–23. doi: 10.1086/324355
261. Marques De Brito CM, Pires MQ, Pacheco RS. Chagas disease and HIV co-infection: genetic analyses of two Trypanosoma cruzi strains under experimental immunosuppression. Kinetoplastid Biol Dis. (2003) 2:17. doi: 10.1186/1475-9292-2-17
262. Marcon GEB, Ferreira JJG, de Almeida EA, Delicio AM, Pereira MB, Wanderley JDS, et al. Parasite load evaluation by qPCR and blood culture in Chagas disease and HIV co-infected patients under antiretroviral therapy. PloS Negl Trop Dis. (2022) 16:e0010317. doi: 10.1371/journal.pntd.0010317
263. Burgos JM, Begher S, Silva HM, Bisio M, Duffy T, Levin MJ, et al. Molecular identification of Trypanosoma cruzi I tropism for central nervous system in Chagas reactivation due to AIDS. Am J Trop Med Hyg. (2008) 78:294–7.
264. Costales JA, Kotton CN, Zurita-Leal AC, Garcia-Perez J, Llewellyn MS, Messenger LA, et al. Chagas disease reactivation in a heart transplant patient infected by domestic Trypanosoma cruzi discrete typing unit I (TcIDOM). Parasit Vectors. (2015) 8:435. doi: 10.1186/s13071-015-1039-3
265. Inga LAC, Olivera MJ. Reactivation of Chagas disease in a heart transplant patient infected by sylvatic Trypanosoma cruzi discrete typing unit I. Rev Soc Bras Med Trop. (2019) 52:e20180512. doi: 10.1590/0037-8682-0512-2018
266. Cura CI, Lucero RH, Bisio M, Oshiro E, Formichelli LB, Burgos JM, et al. Trypanosoma cruzi discrete typing units in Chagas disease patients from endemic and non-endemic regions of Argentina. Parasitology. (2012) 139:516–21. doi: 10.1017/S0031182011002186
267. Silva JS, Barral-Netto M, Reed SG. Aggravation of both Trypanosoma cruzi and murine leukemia virus by concomitant infections. Am J Trop Med Hyg. (1993) 49:589–97. doi: 10.4269/ajtmh.1993.49.589
268. Martins LP, Castanho RE, da Rosa JA, da Silva LC, de Godoy CA, Rosa Rde M. Caracterização biológica, histopatológica e análise de ácido nucléico de uma cepa Trypanosoma cruzi da região de Marília, SP, Brasil. Rev Soc Bras Med Trop. (2003) 36:35–9. doi: 10.1590/s0037-86822003000100006
269. Picado A, Cruz I, Redard-Jacot M, Schijman AG, Torrico F, Sosa-Estani S, et al. The burden of congenital Chagas disease and implementation of molecular diagnostic tools in Latin America. BMJ Glob Health. (2018) 3:e001069. doi: 10.1136/bmjgh-2018-001069
270. Shikanai Yasuda MA. Emerging and reemerging forms of Trypanosoma cruzi transmission. Mem Inst Oswaldo Cruz. (2022) 117:e210033. doi: 10.1590/0074-02760210033
271. Gascon J, Bern C, Pinazo MJ. Chagas disease in Spain, the United States and other non-endemic countries. Acta Trop. (2010) 115:22–7. doi: 10.1016/j.actatropica.2009.07.019
272. Rodari P, Angheben A, Gennati G, Trezzi L, Bargiggia G, Maino M, et al. Congenital Chagas disease in a non-endemic area: Results from a control programme in Bergamo province, Northern Italy. Travel Med Infect Dis. (2018) 25:31–4. doi: 10.1016/j.tmaid.2018.04.011
273. Colombo V, Giacomelli A, Casazza G, Galimberti L, Bonazzetti C, Sabaini F, et al. Trypanosoma cruzi infection in Latin American pregnant women living outside endemic countries and frequency of congenital transmission: a systematic review and meta-analysis. J Travel Med. (2021) 28:taaa170. doi: 10.1093/jtm/taaa170
274. Abras A, Ballart C, Fernández-Arévalo A, Pinazo MJ, Gascón J, Muñoz C, et al. Worldwide Control and Management of Chagas Disease in a New Era of Globalization: a Close Look at Congenital Trypanosoma cruzi Infection. Clin Microbiol Rev. (2022) 35:e0015221. doi: 10.1128/cmr.00152-21
275. Howard EJ, Xiong X, Carlier Y, Sosa-Estani S, Buekens P. Frequency of the congenital transmission of Trypanosoma cruzi: a systematic review and meta-analysis. BJOG. (2014) 121:22–33. doi: 10.1111/1471-0528.12396
276. Burgos JM, Altcheh J, Bisio M, Duffy T, Valadares HM, Seidenstein ME, et al. Direct molecular profiling of minicircle signatures and lineages of Trypanosoma cruzi bloodstream populations causing congenital Chagas disease. Int J Parasitol. (2007) 37:1319–27. doi: 10.1016/j.ijpara.2007.04.015
277. Cencig S, Coltel N, Truyens C, Carlier Y. Fertility, gestation outcome and parasite congenital transmissibility in mice infected with TcI, TcII and TcVI genotypes of Trypanosoma cruzi. PloS Negl Trop Dis. (2013) 7:e2271. doi: 10.1371/journal.pntd.0002271
278. Alkmim-Oliveira SM, Costa-Martins AG, Kappel HB, Correia D, Ramirez LE, Lages-Silva E. Trypanosoma cruzi experimental congenital transmission associated with TcV and TcI subpatent maternal parasitemia. Parasitol Res. (2013) 112:671–8. doi: 10.1007/s00436-012-3184-3
279. Virreira M, Alonso-Vega C, Solano M, Jijena J, Brutus L, Bustamante Z, et al. Congenital chagas disease in Bolivia is not associated with DNA polymorphism of trypanosoma cruzi. Am J Trop Med Hyg. (2006) 75:871–9.
280. Bua J, Volta BJ, Perrone AE, Scollo K, Velázquez EB, Ruiz AM, et al. How to improve the early diagnosis of Trypanosoma cruzi infection: relationship between validated conventional diagnosis and quantitative DNA amplification in congenitally infected children. PloS Negl Trop Dis. (2013) 7:e2476. doi: 10.1371/journal.pntd.0002476
281. Garcia A, Ortiz S, Iribarren C, Bahamonde MI, Solari A. Congenital co-infection with different Trypanosoma cruzi lineages. Parasitol Int. (2014) 63:138–9.
282. Hakim JMC, Waltmann A, Tinajeros F, Kharabora O, Machaca EM, Calderon M, et al. Amplicon sequencing reveals complex infection in infants congenitally infected with trypanosoma cruzi and informs the dynamics of parasite transmission. J Infect Dis. (2023) 228:769–76. doi: 10.1093/infdis/jiad125
283. Burgos JM, Altcheh J, Petrucelli N, Bisio M, Levin MJ, Freilij H, et al. Molecular diagnosis and treatment monitoring of congenital transmission of Trypanosoma cruzi to twins of a triplet delivery. Diagn Microbiol Infect Dis. (2009) 65:58–61. doi: 10.1016/j.diagmicrobio.2009.04.010
284. Corrales RM, Mora MC, Negrette OS, Diosque P, Lacunza D, Virreira M, et al. Congenital Chagas disease involves Trypanosoma cruzi sub-lineage IId in the northwestern province of Salta, Argentina. Infect Genet Evol. (2009) 9:278–82. doi: 10.1016/j.meegid.2008.12.008
285. Bisio M, Seidenstein ME, Burgos JM, Ballering G, Risso M, Pontoriero R, et al. Urbanization of congenital transmission of Trypanosoma cruzi: prospective polymerase chain reaction study in pregnancy. Trans R Soc Trop Med Hyg. (2011) 105:543–9. doi: 10.1016/j.trstmh.2011.07.003
286. Ortiz S, Zulantay I, Solari A, Bisio M, Schijman A, Carlier Y, et al. Presence of Trypanosoma cruzi in pregnant women and typing of lineages in congenital cases. Acta Trop. (2012) 124:243–6. doi: 10.1016/j.actatropica.2012.08.001
287. Falla A, Herrera C, Fajardo A, Montilla M, Vallejo GA, Guhl F. Haplotype identification within Trypanosoma cruzi I in Colombian isolates from several reservoirs, vectors and humans. Acta Trop. (2009) 110:15–21. doi: 10.1016/j.actatropica.2008.12.003
288. Villanueva-Lizama L, Teh-Poot C, Majeau A, Herrera C, Dumonteil E. Molecular genotyping of trypanosoma cruzi by next-generation sequencing of the mini-exon gene reveals infections with multiple parasite discrete typing units in chagasic patients from Yucatan, Mexico. J Infect Dis. (2019) 219:1980–8. doi: 10.1093/infdis/jiz047
289. Herrera C, Truyens C, Dumonteil E, Alger J, Sosa-Estani S, Cafferata ML, et al. Phylogenetic analysis of trypanosoma cruzi from pregnant women and newborns from Argentina, Honduras, and Mexico suggests an association of parasite haplotypes with congenital transmission of the parasite. J Mol Diagn. (2019) 21:1095–105. doi: 10.1016/j.jmoldx.2019.07.004
290. Llewellyn MS, Messenger LA, Luquetti AO, Garcia L, Torrico F, Tavares SB, et al. Deep sequencing of the Trypanosoma cruzi GP63 surface proteases reveals diversity and diversifying selection among chronic and congenital Chagas disease patients. PloS Negl Trop Dis. (2015) 9:e0003458. doi: 10.1371/journal.pntd.0003458
291. Martins-Melo FR, Lima Mda S, Ramos AN Jr, Alencar CH, Heukelbach J. Prevalence of Chagas disease in pregnant women and congenital transmission of Trypanosoma cruzi in Brazil: a systematic review and meta-analysis. Trop Med Int Health. (2014) 19:943–57. doi: 10.1111/tmi.12328
292. Bittencourt AL, Mota E, Povoa M. Isoenzyme characterization of Trypanosoma cruzi from congenital cases of Chagas’ disease. Ann Trop Med Parasitol. (1985) 79:393–6. doi: 10.1080/00034983.1985
293. Freitas VLT, Piotto MR, Esper HR, Nakanishi EYS, Fonseca CA, Assy JGPL, et al. Detection of Trypanosoma cruzi DTUs TcI and TcIV in two outbreaks of orally-transmitted Chagas disease in the Northern region of Brazil. Rev Inst Med Trop Sao Paulo. (2023) 65:e7. doi: 10.1590/S1678-9946202365007
294. Torrico F, Vega CA, Suarez E, Tellez T, Brutus L, Rodriguez P, et al. Are maternal re-infections with Trypanosoma cruzi associated with higher morbidity and mortality of congenital Chagas disease? Trop Med Int Health. (2006) 11:628–35. doi: 10.1111/j.1365-3156.2006.01623.x
295. Carlier Y, Truyens C. Congenital Chagas disease as an ecological model of interactions between Trypanosoma cruzi parasites, pregnant women, placenta and fetuses. Acta Trop. (2015) 151:103–15. doi: 10.1016/j.actatropica.2015.07.016
296. Juiz NA, Cayo NM, Burgos M, Salvo ME, Nasser JR, Búa J, et al. Human polymorphisms in placentally expressed genes and their association with susceptibility to congenital trypanosoma cruzi infection. J Infect Dis. (2016) 213:1299–306. doi: 10.1093/infdis/jiv561
297. Kemmerling U, Osuna A, Schijman AG, Truyens C. Congenital transmission of trypanosoma cruzi: A review about the interactions between the parasite, the placenta, the maternal and the fetal/neonatal immune responses. Front Microbiol. (2019) 10:1854. doi: 10.3389/fmicb.2019.01854
298. Klein MD, Proaño A, Noazin S, Sciaudone M, Gilman RH, Bowman NM. Risk factors for vertical transmission of Chagas disease: A systematic review and meta-analysis. Int J Infect Dis. (2021) 105:357–73. doi: 10.1016/j.ijid.2021.02.074
299. Andrade SG. The influence of the strain of Trypanosoma cruzi in placental infections in mice. Trans R Soc Trop Med Hyg. (1982) 76:123–8. doi: 10.1016/0035-9203(82)90036-0
300. Medina L, Castillo C, Liempi A, Herbach M, Cabrera G, Valenzuela L, et al. Differential infectivity of two Trypanosoma cruzi strains in placental cells and tissue. Acta Trop. (2018) 186:35–40. doi: 10.1016/j.actatropica.2018.07.001
301. Castillo C, Muñoz L, Carrillo I, Liempi A, Medina L, Galanti N, et al. Toll-like receptor-2 mediates local innate immune response against Trypanosoma cruzi in ex vivo infected human placental chorionic villi explants. Placenta. (2017) 60:40–6. doi: 10.1016/j.placenta.2017.10.005
302. Castillo C, Carrillo I, Libisch G, Juiz N, Schijman A, Robello C, et al. Host-parasite interaction: changes in human placental gene expression induced by Trypanosoma cruzi. Parasit Vectors. (2018) 11:479. doi: 10.1186/s13071-018-2988-0
303. Juiz NA, Torrejón I, Burgos M, Torres AMF, Duffy T, Cayo NM, et al. Alterations in placental gene expression of pregnant women with chronic chagas disease. Am J Pathol. (2018) 188:1345–53. doi: 10.1016/j.ajpath.2018.02.011
304. Liempi A, Castillo C, Carrillo I, Muñoz L, Droguett D, Galanti N, et al. A local innate immune response against Trypanosoma cruzi in the human placenta: The epithelial turnover of the trophoblast. Microb Pathog. (2016) 99:123–9. doi: 10.1016/j.micpath.2016.08.022
305. Juiz NA, Solana ME, Acevedo GR, Benatar AF, Ramirez JC, da Costa PA, et al. Different genotypes of Trypanosoma cruzi produce distinctive placental environment genetic response in chronic experimental infection. PloS Negl Trop Dis. (2017) 11:e0005436. doi: 10.1371/journal.pntd.0005436
306. Coura RJ, Castro SL. A critical review on Chagas disease chemotherapy. Mem Inst Oswaldo Cruz. (2002) 97:3–24. doi: 10.1590/s0074-02762002000100001
307. Filardi LS, Brener Z. A rapid method for testing in vivo the susceptibility of different strains of Trypanosoma cruzi to active chemotherapeutic agents. Mem Inst Oswaldo Cruz. (1984) 79:221–5. doi: 10.1590/s0074-02761984000200008
308. Molina I, Salvador F, Sánchez-Montalvá A. Posaconazole versus benznidazole for chronic Chagas’ disease. N Engl J Med. (2014) 371:966. doi: 10.1056/NEJMc1407914
309. Morillo CA, Marin-Neto JA, Avezum A, Sosa-Estani S, Rassi A Jr, Rosas F, et al. Randomized trial of benznidazole for chronic chagas’ Cardiomyopathy. N Engl J Med. (2015) 373:1295–306. doi: 10.1056/NEJMoa1507574
310. Lascano F, García Bournissen F, Altcheh J. Review of pharmacological options for the treatment of Chagas disease. Br J Clin Pharmacol. (2022) 88:383–402. doi: 10.1111/bcp.14700
311. Bahia-Oliveira LM, Gomes JA, Cançado JR, Ferrari TC, Lemos EM, Luz ZM, et al. Immunological and clinical evaluation of chagasic patients subjected to chemotherapy during the acute phase of Trypanosoma cruzi infection 14-30 years ago. J Infect Dis. (2000) 182:634–8. doi: 10.1086/315743
312. de Andrade AL, Zicker F, de Oliveira RM, Almeida Silva S, Luquetti A, Travassos LR, et al. Randomised trial of efficacy of benznidazole in treatment of early Trypanosoma cruzi infection. Lancet. (1996) 348:1407–13. doi: 10.1016/s0140-6736(96)04128-1
313. Sosa Estani S, Segura EL, Ruiz AM, Velazquez E, Porcel BM, Yampotis C. Efficacy of chemotherapy with benznidazole in children in the indeterminate phase of Chagas’ disease. Am J Trop Med Hyg. (1998) 59:526–9. doi: 10.4269/ajtmh.1998.59.526
314. Sosa-Estani S, Cura E, Velazquez E, Yampotis C, Segura EL. Etiological treatment of young women infected with Trypanosoma cruzi, and prevention of congenital transmission. Rev Soc Bras Med Trop. (2009) 42:484–7. doi: 10.1590/s0037-86822009000500002
315. Yun O, Lima MA, Ellman T, Chambi W, Castillo S, Flevaud L, et al. Feasibility, drug safety, and effectiveness of etiological treatment programs for Chagas disease in Honduras, Guatemala, and Bolivia: 10-year experience of Médecins Sans Frontières. PloS Negl Trop Dis. (2009) 3:e488. doi: 10.1371/journal.pntd.0000488
316. Cancado JR. Long term evaluation of etiological treatment of chagas disease with benznidazole. Rev Inst Med Trop Sao Paulo. (2002) 44:29–37.
317. Dias JC. Chagas disease: successes and challenges. Cad Saude Publica. (2006) 22:2020–1. doi: 10.1590/s0102-311x2006001000001
318. Guedes PM, Silva GK, Gutierrez FR, Silva JS. Current status of Chagas disease chemotherapy. Expert Rev Anti Infect Ther. (2011) 9:609–20. doi: 10.1586/eri.11.31
319. Fernández ML, Marson ME, Ramirez JC, Mastrantonio G, Schijman AG, Altcheh J, et al. Pharmacokinetic and pharmacodynamic responses in adult patients with Chagas disease treated with a new formulation of benznidazole. Mem Inst Oswaldo Cruz. (2016) 111:218–21. doi: 10.1590/0074-02760150401
320. Lauria-Pires L, Braga MS, Vexenat AC, Nitz N, Simões-Barbosa A, Tinoco DL, et al. Progressive chronic Chagas heart disease ten years after treatment with anti-Trypanosoma cruzi nitroderivatives. Am J Trop Med Hyg. (2000) 63:111–8. doi: 10.4269/ajtmh.2000.63.111
321. Viotti R, Vigliano C, Lococo B, Bertocchi G, Petti M, Alvarez MG, et al. Long-term cardiac outcomes of treating chronic Chagas disease with benznidazole versus no treatment: a nonrandomized trial. Ann Intern Med. (2006) 144:724–34. doi: 10.7326/0003-4819-144-10-200605160-00006
322. Marin-Neto JA, Rassi A Jr, Morillo CA, Avezum A, Connolly SJ, Sosa-Estani S, et al. Rationale and design of a randomized placebo-controlled trial assessing the effects of etiologic treatment in Chagas’ cardiomyopathy: the BENznidazole Evaluation For Interrupting Trypanosomiasis (BENEFIT). Am Heart J. (2008) 156:37–43. doi: 10.1016/j.ahj.2008.04.001
323. Coura JR, Borges-Pereira J. Chronic phase of Chagas disease: why should it be treated? A comprehensive review. Mem Inst Oswaldo Cruz. (2011) 106:641–5. doi: 10.1590/s0074-02762011000600001
324. Filardi LS, Brener Z. Susceptibility and natural resistance of Trypanosoma cruzi strains to drugs used clinically in Chagas disease. Trans R Soc Trop Med Hyg. (1987) 81:755–9. doi: 10.1016/0035-9203(87)90020-4
325. Tibayrenc M, Ayala FJ. Isozyme variability in trypanosoma cruzi, the agent of chagas’ disease: genetical, taxonomical, and epidemiological significance. Evolution. (1988) 42:277–92. doi: 10.1111/j.1558-5646.1988.tb04132.x
326. Andrade SG, Rassi A, Magalhaes JB, Ferriolli Filho F, Luquetti AO. Specific chemotherapy of Chagas disease: a comparison between the response in patients and experimental animals inoculated with the same strains. Trans R Soc Trop Med Hyg. (1992) 86:624–6. doi: 10.1016/0035-9203(92)90156-7
327. Guedes PM, Urbina JA, de Lana M, Afonso LC, Veloso VM, Tafuri WL, et al. Activity of the new triazole derivative albaconazole against Trypanosoma (Schizotrypanum) cruzi in dog hosts. Antimicrob Agents Chemother. (2004) 48:4286–92. doi: 10.1128/AAC.48.11.4286-4292.2004
328. Toledo MJ, Guilherme AL, da Silva JC, de Gasperi MV, Mendes AP, Gomes ML, et al. Trypanosoma cruzi: chemotherapy with benznidazole in mice inoculated with strains from Paraná state and from different endemic areas of Brazil. Rev Inst Med Trop Sao Paulo. (1997) 39:283–90. doi: 10.1590/s0036-46651997000500007
329. Brener Z, Costa CA, Chiari C. Differences in the susceptibility of Trypanosoma cruzi strains to active chemotherapeutic agents. Rev Inst Med Trop Sao Paulo. (1976) 18:450–5.
330. Andrade SG, Magalhães JB, Pontes AL. Terapêutica da fase crônica da infeccao experimental pelo Trypanosoma cruzi com o benzonidazol e o nifurtimox [Therapy of the chronic phase of the experimental infection by Trypanosoma cruzi with benzonidazole and nifurtimox]. Rev Soc Bras Med Trop. (1989) 22:113–8. doi: 10.1590/s0037-86821989000300001
331. Guedes PM, Veloso VM, Tafuri WL, Galvão LM, Carneiro CM, Md L, et al. The dog as model for chemotherapy of the Chagas’ disease. Acta Trop. (2002) 84:9–17. doi: 10.1016/s0001-706x(02)00139-0
332. Bianchi F, Cucunubá Z, Guhl F, González NL, Freilij H, Nicholls RS, et al. Follow-up of an asymptomatic Chagas disease population of children after treatment with nifurtimox (Lampit) in a sylvatic endemic transmission area of Colombia. PloS Negl Trop Dis. (2015) 9:e0003465. doi: 10.1371/journal.pntd.0003465
333. Toledo MJ, Bahia MT, Veloso VM, Carneiro CM, MaChado-Coelho GL, Alves CF, et al. Effects of specific treatment on parasitological and histopathological parameters in mice infected with different Trypanosoma cruzi clonal genotypes. J Antimicrob Chemother. (2004) 53:1045–53. doi: 10.1093/jac/dkh224
334. Revollo S, Oury B, Vela A, Tibayrenc M, Sereno D. In vitro benznidazole and nifurtimox susceptibility profile of trypanosoma cruzi strains belonging to discrete typing units TcI, TcII, and TcV. Pathogens. (2019) 8:197. doi: 10.3390/pathogens8040197
335. Murta SM, Gazzinelli RT, Brener Z, Romanha AJ. Molecular characterization of susceptible and naturally resistant strains of Trypanosoma cruzi to benznidazole and nifurtimox. Mol Biochem Parasitol. (1998) 93:203–14. doi: 10.1016/s0166-6851(98)00037-1
336. Villarreal D, Barnabé C, Sereno D, Tibayrenc M. Lack of correlation between in vitro susceptibility to Benznidazole and phylogenetic diversity of Trypanosoma cruzi, the agent of Chagas disease. Exp Parasitol. (2004) 108:24–31. doi: 10.1016/j.exppara.2004.07.001
337. Moreno M, D’ávila DA, Silva MN, Galvão LM, Macedo AM, Chiari E, et al. Trypanosoma cruzi benznidazole susceptibility in vitro does not predict the therapeutic outcome of human Chagas disease. Mem Inst Oswaldo Cruz. (2010) 105:918–24. doi: 10.1590/s0074-02762010000700014
338. Gruendling AP, Massago M, Teston AP, Monteiro WM, Kaneshima EN, Araújo SM, et al. Impact of benznidazole on infection course in mice experimentally infected with Trypanosoma cruzi I, II, and IV. Am J Trop Med Hyg. (2015) 92:1178–89. doi: 10.4269/ajtmh.13-0690
339. Herrera C, Guhl F, Falla A, Fajardo A, Montilla M, Adolfo Vallejo G, et al. Genetic Variability and Phylogenetic Relationships within Trypanosoma cruzi I Isolated in Colombia Based on Miniexon Gene Sequences. J Parasitol Res. (2009) 2009:897364. doi: 10.1155/2009/897364
340. Llewellyn MS, Miles MA, Carrasco HJ, Lewis MD, Yeo M, Vargas J, et al. Genome-scale multilocus microsatellite typing of Trypanosoma cruzi discrete typing unit I reveals phylogeographic structure and specific genotypes linked to human infection. PloS Pathog. (2009) 5:e1000410. doi: 10.1371/journal.ppat.1000410
341. Valente SA, da Costa Valente V, das Neves Pinto AY, de Jesus Barbosa César M, dos Santos MP, Miranda CO, et al. Analysis of an acute Chagas disease outbreak in the Brazilian Amazon: human cases, triatomines, reservoir mammals and parasites. Trans R Soc Trop Med Hyg. (2009) 103:291–7. doi: 10.1016/j.trstmh.2008.10.047
342. Pinto AY, Ferreira AG Jr, Valente Vda C, Harada GS, Valente SA. Urban outbreak of acute Chagas disease in Amazon region of Brazil: four-year follow-up after treatment with benznidazole. Rev Panam Salud Publica. (2009) 25:77–83. doi: 10.1590/s1020-49892009000100012
343. Oliveira-Silva JC, MaChado-de-Assis GF, Oliveira MT, Paiva NC, Araújo MS, Carneiro CM, et al. Experimental benznidazole treatment of Trypanosoma cruzi II strains isolated from children of the Jequitinhonha Valley, Minas Gerais, Brazil, with Chagas disease. Mem Inst Oswaldo Cruz. (2015) 110:86–94. doi: 10.1590/0074-02760140260
344. Molina J, Martins-Filho O, Brener Z, Romanha AJ, Loebenberg D, Urbina JA. Activities of the triazole derivative SCH 56592 (posaconazole) against drug-resistant strains of the protozoan parasite Trypanosoma (Schizotrypanum) cruzi in immunocompetent and immunosuppressed murine hosts. Antimicrob Agents Chemother. (2000) 44:150–5. doi: 10.1128/AAC.44.1.150-155.2000
345. Moraes CB, Giardini MA, Kim H, Franco CH, Araujo-Junior AM, Schenkman S, et al. Nitroheterocyclic compounds are more efficacious than CYP51 inhibitors against Trypanosoma cruzi: implications for Chagas disease drug discovery and development. Sci Rep. (2014) 4:4703. doi: 10.1038/srep04703
346. Urbina JA, Payares G, Contreras LM, Liendo A, Sanoja C, Molina J, et al. Antiproliferative effects and mechanism of action of SCH 56592 against Trypanosoma (Schizotrypanum) cruzi: in vitro and in vivo studies. Antimicrob Agents Chemother. (1998) 42:1771–7. doi: 10.1128/AAC.42.7.1771
347. Urbina JA, Payares G, Sanoja C, Molina J, Lira R, Brener Z, et al. Parasitological cure of acute and chronic experimental Chagas disease using the long-acting experimental triazole TAK-187. Activity against drug-resistant Trypanosoma cruzi strains. Int J Antimicrob Agents. (2003) 21:39–48. doi: 10.1016/s0924-8579(02)00274-1
348. Ferraz ML, Gazzinelli RT, Alves RO, Urbina JA, Romanha AJ. The Anti-Trypanosoma cruzi activity of posaconazole in a murine model of acute Chagas’ disease is less dependent on gamma interferon than that of benznidazole. Antimicrob Agents Chemother. (2007) 51:1359–64. doi: 10.1128/AAC.01170-06
349. Olivieri BP, Molina JT, de Castro SL, Pereira MC, Calvet CM, Urbina JA, et al. A comparative study of posaconazole and benznidazole in the prevention of heart damage and promotion of trypanocidal immune response in a murine model of Chagas disease. Int J Antimicrob Agents. (2010) 36:79–83. doi: 10.1016/j.ijantimicag.2010.03.006
350. Veiga-Santos P, Barrias ES, Santos JF, de Barros Moreira TL, de Carvalho TM, Urbina JA, et al. Effects of amiodarone and posaconazole on the growth and ultrastructure of Trypanosoma cruzi. Int J Antimicrob Agents. (2012) 40:61–71. doi: 10.1016/j.ijantimicag.2012.03.009
351. Bahia MT, de Andrade IM, Martins TA, do Nascimento ÁF, Diniz Lde F, Caldas IS, et al. Fexinidazole: a potential new drug candidate for Chagas disease. PloS Negl Trop Dis. (2012) 6:e1870. doi: 10.1371/journal.pntd.0001870
352. Bustamante JM, Craft JM, Crowe BD, Ketchie SA, Tarleton RL. New, combined, and reduced dosing treatment protocols cure Trypanosoma cruzi infection in mice. J Infect Dis. (2014) 209:150–62. doi: 10.1093/infdis/jit420
353. Bosseno MF, Yacsik N, Vargas F, Brenière SF. Selection of Trypanosoma cruzi clonal genotypes (clonet 20 and 39) isolated from Bolivian triatomines following subculture in liquid medium. Mem Inst Oswaldo Cruz. (2000) 95:601–7. doi: 10.1590/s0074-02762000000500002
354. Solari A, Campillay R, Ortíz S, Wallace A. Identification of Trypanosoma cruzi genotypes circulating in Chilean chagasic patients. Exp Parasitol. (2001) 97:226–33. doi: 10.1006/expr.2001.4607
355. Deane MP, Mangia RH, Pereira NM, Momen H, Gonçalves AM, Morel CM. Trypanosoma cruzi: strain selection by different schedules of mouse passage of an initially mixed infection. Mem Inst Oswaldo Cruz. (1984) 79:495–7. doi: 10.1590/s0074-02761984000400016
356. Canavaci AM, Bustamante JM, Padilla AM, Perez Brandan CM, Simpson LJ, Xu D, et al. In vitro and in vivo high-throughput assays for the testing of anti-Trypanosoma cruzi compounds. PloS Negl Trop Dis. (2010) 4:e740. doi: 10.1371/journal.pntd.0000740
357. Alessio GD, Côrtes DF, MaChado de Assis GF, Júnior PA, Ferro EA, Antonelli LR, et al. Innovations in diagnosis and post-therapeutic monitoring of Chagas disease: Simultaneous flow cytometric detection of IgG1 antibodies anti-live amastigote, anti-live trypomastigote, and anti-fixed epimastigote forms of Trypanosoma cruzi. J Immunol Methods. (2014) 413:32–44. doi: 10.1016/j.jim.2014.07.005
358. Sanchez-Valdez F, Padilla ÁM, Bustamante JM, Hawkins CWD, Tarleton RL. Quantitative 3D imaging of trypanosoma cruzi-infected cells, dormant amastigotes, and T cells in intact clarified organs. J Vis Exp. (2022) 184. doi: 10.3791/63919
359. Desale H, Herrera C, Dumonteil E. Trypanosoma cruzi amastigote transcriptome analysis reveals heterogenous populations with replicating and dormant parasites. Microbes Infect. (2023) 20:105240. doi: 10.1016/j.micinf.2023.105240
360. Bustamante JM, Sanchez-Valdez F, Padilla AM, White B, Wang W, Tarleton RL. A modified drug regimen clears active and dormant trypanosomes in mouse models of Chagas disease. Sci Transl Med. (2020) 12:eabb7656. doi: 10.1126/scitranslmed.abb7656
361. Camandaroba EL, Reis EA, Gonçalves MS, Reis MG, Andrade SG. Trypanosoma cruzi: susceptibility to chemotherapy with benznidazole of clones isolated from the highly resistant Colombian strain. Rev Soc Bras Med Trop. (2003) 36:201–9. doi: 10.1590/s0037-86822003000200002
362. Bhattacharyya T, Brooks J, Yeo M, Carrasco HJ, Lewis MD, Llewellyn MS, et al. Analysis of molecular diversity of the Trypanosoma cruzi trypomastigote small surface antigen reveals novel epitopes, evidence of positive selection and potential implications for lineage-specific serology. Int J Parasitol. (2010) 40:921–8. doi: 10.1016/j.ijpara.2010.01.002
363. Mendes TA, Reis Cunha JL, de Almeida Lourdes R, Rodrigues Luiz GF, Lemos LD, dos Santos AR, et al. Identification of strain-specific B-cell epitopes in Trypanosoma cruzi using genome-scale epitope prediction and high-throughput immunoscreening with peptide arrays. PloS Negl Trop Dis. (2013) 7:e2524. doi: 10.1371/journal.pntd.0002524
364. Bhattacharyya T, Falconar AK, Luquetti AO, Costales JA, Grijalva MJ, Lewis MD, et al. Development of peptide-based lineage-specific serology for chronic Chagas disease: geographical and clinical distribution of epitope recognition. PloS Negl Trop Dis. (2014) 8:e2892. doi: 10.1371/journal.pntd.0002892
365. Bhattacharyya T, Mills EA, Jansen AM, Miles MA. Prospects for T. cruzi lineage-specific serological surveillance of wild mammals. Acta Trop. (2015) 151:182–6. doi: 10.1016/j.actatropica.2015.06.017
366. Kerr CL, Bhattacharyya T, Xavier SC, Barros JH, Lima VS, Jansen AM, et al. Lineage-specific serology confirms Brazilian Atlantic forest lion tamarins, Leontopithecus chrysomelas and Leontopithecus rosalia, as reservoir hosts of Trypanosoma cruzi II (TcII). Parasit Vectors. (2016) 9:584. doi: 10.1186/s13071-016-1873-y
367. Alessio GD, de Araújo FF, Côrtes DF, Sales Júnior PA, Lima DC, Gomes MS, et al. Performance of TcI/TcVI/TcII Chagas-Flow ATE-IgG2a for universal and genotype-specific serodiagnosis of Trypanosoma cruzi infection. PloS Negl Trop Dis. (2017) 11:e0005444. doi: 10.1371/journal.pntd.0005444
368. Alessio GD, de Araújo FF, Sales Júnior PA, Gomes MS, Amaral LRD, Pascoal Xavier MA, et al. Accomplishing the genotype-specific serodiagnosis of single and dual Trypanosoma cruzi infections by flow cytometry Chagas-Flow ATE-IgG2a. PloS Negl Trop Dis. (2018) 12:e0006140. doi: 10.1371/journal.pntd.0006140
369. Bhattacharyya T, Messenger LA, Bern C, Mertens P, Gilleman Q, Zeippen N, et al. Severity of chagasic cardiomyopathy is associated with response to a novel rapid diagnostic test for trypanosoma cruzi TcII/V/VI. Clin Infect Dis. (2018) 67:519–24. doi: 10.1093/cid/ciy121
370. Bhattacharyya T, Murphy N, Miles MA. Trypanosoma cruzi lineage-specific serology: new rapid tests for resolving clinical and ecological associations. Future Sci OA. (2019) 5:FSO422. doi: 10.2144/fsoa-2019-0103
371. Alessio GD, de Araújo FF, Silva JS, Júnior PAS, de Souza Gomes M, do Amaral LR, et al. Human Chagas-Flow ATE-IgG1 for advanced universal and Trypanosoma cruzi Discrete Typing Units-specific serodiagnosis of Chagas disease. Sci Rep. (2020) 10:13296. doi: 10.1038/s41598-020-69921-z
372. Lages-Silva E, Ramírez LE, Pedrosa AL, Crema E, da Cunha Galvão LM, Pena SD, et al. Variability of kinetoplast DNA gene signatures of Trypanosoma cruzi II strains from patients with different clinical forms of Chagas’ disease in Brazil. J Clin Microbiol. (2006) 44:2167–71. doi: 10.1128/JCM.02124-05
373. Umezawa ES, Bastos SF, Camargo ME, Yamauchi LM, Santos MR, Gonzalez A, et al. Evaluation of recombinant antigens for serodiagnosis of Chagas’ disease in South and Central America. J Clin Microbiol. (1999) 37:1554–60. doi: 10.1128/JCM.37.5.1554-1560.1999
374. Umezawa ES, Luquetti AO, Levitus G, Ponce C, Ponce E, Henriquez D, et al. Serodiagnosis of chronic and acute Chagas’ disease with Trypanosoma cruzi recombinant proteins: results of a collaborative study in six Latin American countries. J Clin Microbiol. (2004) 42:449–52. doi: 10.1128/JCM.42.1.449-452.2004
375. Verani JR, Seitz A, Gilman RH, LaFuente C, Galdos-Cardenas G, Kawai V, et al. Geographic variation in the sensitivity of recombinant antigen-based rapid tests for chronic Trypanosoma cruzi infection. Am J Trop Med Hyg. (2009) 80:410–5.
376. Reis-Cunha JL, Mendes TA, de Almeida Lourdes R, Ribeiro DR, MaChado-de-Avila RA, de Oliveira Tavares M, et al. Genome-wide screening and identification of new Trypanosoma cruzi antigens with potential application for chronic Chagas disease diagnosis. PloS One. (2014) 9:e106304. doi: 10.1371/journal.pone.0106304
377. Murthy VK, Dibbern KM, Campbell DA. PCR amplification of mini-exon genes differentiates Trypanosoma cruzi from Trypanosoma rangeli. Mol Cell Probes. (1992) 6:237–43. doi: 10.1016/0890-8508(92)90022-p
378. Souto RP, Zingales B. Sensitive detection and strain classification of Trypanosoma cruzi by amplification of a ribosomal RNA sequence. Mol Biochem Parasitol. (1993) 62:45–52. doi: 10.1016/0166-6851(93)90176-x
379. Barnabé C, Brisse S, Tibayrenc M. Population structure and genetic typing of Trypanosoma cruzi, the agent of Chagas disease: a multilocus enzyme electrophoresis approach. Parasitology. (2000) 120:513–26. doi: 10.1017/s0031182099005661
380. Lauthier JJ, Tomasini N, Barnabé C, Rumi MM, D’Amato AM, Ragone PG, et al. Candidate targets for Multilocus Sequence Typing of Trypanosoma cruzi: validation using parasite stocks from the Chaco Region and a set of reference strains. Infect Genet Evol. (2012) 12:350–8. doi: 10.1016/j.meegid.2011.12.008
381. Diosque P, Tomasini N, Lauthier JJ, Messenger LA, Monje Rumi MM, Ragone PG, et al. Optimized multilocus sequence typing (MLST) scheme for Trypanosoma cruzi. PloS Negl Trop Dis. (2014) 8:e3117. doi: 10.1371/journal.pntd.0003117
382. Tomasini N, Lauthier JJ, Monje Rumi MM, Ragone PG, Alberti D’Amato AM, Brandán CP, et al. Preponderant clonal evolution of Trypanosoma cruzi I from Argentinean Chaco revealed by Multilocus Sequence Typing (MLST). Infect Genet Evol. (2014) 27:348–54. doi: 10.1016/j.meegid.2014.08.003
383. Bastrenta B, Bosseno MF, Barnabé C, Tibayrenc M, Brenière SF. Restriction fragment length polymorphism of 195 bp repeated satellite DNA of Trypanosoma cruzi supports the existence of two phylogenetic groups. Mem Inst Oswaldo Cruz. (1999) 94:323–8. doi: 10.1590/s0074-02761999000300008
384. Cosentino RO, Agüero F. A simple strain typing assay for Trypanosoma cruzi: discrimination of major evolutionary lineages from a single amplification product. PloS Negl Trop Dis. (2012) 6:e1777. doi: 10.1371/journal.pntd.0001777
385. Steindel M, Dias Neto E, Pinto CJ, Grisard EC, Menezes CL, Murta SM, et al. Randomly amplified polymorphic DNA (RAPD) and isoenzyme analysis of Trypanosoma rangeli strains. J Eukaryot Microbiol. (1994) 41:261–7. doi: 10.1111/j.1550-7408.1994.tb01506.x
386. Oliveira RP, Broude NE, Macedo AM, Cantor CR, Smith CL, Pena SD. Probing the genetic population structure of Trypanosoma cruzi with polymorphic microsatellites. Proc Natl Acad Sci U.S.A. (1998) 95:3776–80. doi: 10.1073/pnas.95.7.3776
387. Macedo AM, Pimenta JR, Aguiar RS, Melo AI, Chiari E, Zingales B, et al. Usefulness of microsatellite typing in population genetic studies of Trypanosoma cruzi. Mem Inst Oswaldo Cruz. (2001) 96:407–13. doi: 10.1590/s0074-02762001000300023
388. Messenger LA, Llewellyn MS, Bhattacharyya T, Franzén O, Lewis MD, Ramírez JD, et al. Multiple mitochondrial introgression events and heteroplasmy in trypanosoma cruzi revealed by maxicircle MLST and next generation sequencing. PloS Negl Trop Dis. (2012) 6:e1584. doi: 10.1371/journal.pntd.0001584
389. Solari A, Venegas J, Gonzalez E, Vasquez C. Detection and classification of Trypanosoma cruzi by DNA hybridization with nonradioactive probes. J Protozool. (1991) 38:559–65. doi: 10.1111/j.1550-7408.1991.tb06080.x
390. Breniere SF, Bosseno MF, Revollo S, Rivera MT, Carlier Y, Tibayrenc M. Direct identification of Trypanosoma cruzi natural clones in vectors and mammalian hosts by polymerase chain reaction amplification. Am J Trop Med Hyg. (1992) 46:335–41. doi: 10.4269/ajtmh.1992.46.335
391. Venegas J, Coñoepan W, Pichuantes S, Miranda S, Jercic MI, Gajardo M, et al. Phylogenetic analysis of microsatellite markers further supports the two hybridization events hypothesis as the origin of the Trypanosoma cruzi lineages. Parasitol Res. (2009) 105:191–9. doi: 10.1007/s00436-009-1386-0
392. Rumi MM, Pérez Brandán C, Gil JF, D’Amato AM, Ragone PG, Lauthier JJ, et al. Benznidazole treatment in chronic children infected with Trypanosoma cruzi: serological and molecular follow-up of patients and identification of Discrete Typing Units. Acta Trop. (2013) 128:130–6. doi: 10.1016/j.actatropica.2013.07.003
393. Tibayrenc M, Neubauer K, Barnabé C, Guerrini F, Skarecky D, Ayala FJ. Genetic characterization of six parasitic protozoa: parity between random-primer DNA typing and multilocus enzyme electrophoresis. Proc Natl Acad Sci U.S.A. (1993) 90:1335–9. doi: 10.1073/pnas.90.4.1335
394. Messenger LA, Yeo M, Lewis MD, Llewellyn MS, Miles MA. Molecular genotyping of Trypanosoma cruzi for lineage assignment and population genetics. Methods Mol Biol. (2015) 1201:297–337. doi: 10.1007/978-1-4939-1438-8_19
395. Freitas JM, Lages-Silva E, Crema E, Pena SD, Macedo AM. Real time PCR strategy for the identification of major lineages of Trypanosoma cruzi directly in chronically infected human tissues. Int J Parasitol. (2005) 35:411–7. doi: 10.1016/j.ijpara.2004.10.023
396. Valadares HM, Pimenta JR, de Freitas JM, Duffy T, Bartholomeu DC, Oliveira Rde P, et al. Genetic profiling of Trypanosoma cruzi directly in infected tissues using nested PCR of polymorphic microsatellites. Int J Parasitol. (2008) 38:839–50. doi: 10.1016/j.ijpara.2007.10.017
397. Piron M, Fisa R, Casamitjana N, López-Chejade P, Puig L, Vergés M, et al. Development of a real-time PCR assay for Trypanosoma cruzi detection in blood samples. Acta Trop. (2007) 103:195–200. doi: 10.1016/j.actatropica.2007.05.019
398. Duffy T, Bisio M, Altcheh J, Burgos JM, Diez M, Levin MJ, et al. Accurate real-time PCR strategy for monitoring bloodstream parasitic loads in chagas disease patients. PloS Negl Trop Dis. (2009) 3:e419. doi: 10.1371/journal.pntd.0000419
399. Moreira OC, Ramírez JD, Velázquez E, Melo MF, Lima-Ferreira C, Guhl F, et al. Towards the establishment of a consensus real-time qPCR to monitor Trypanosoma cruzi parasitemia in patients with chronic Chagas disease cardiomyopathy: a substudy from the BENEFIT trial. Acta Trop. (2013) 125:23–31. doi: 10.1016/j.actatropica.2012.08.020
400. Cura CI, Duffy T, Lucero RH, Bisio M, Péneau J, Jimenez-Coello M, et al. Multiplex real-time PCR assay using TaqMan probes for the identification of trypanosoma cruzi DTUs in biological and clinical samples. PloS Negl Trop Dis. (2015) 9:e0003765. doi: 10.1371/journal.pntd.0003765
401. Muñoz-San Martín C, Apt W, Zulantay I. Real-time PCR strategy for the identification of Trypanosoma cruzi discrete typing units directly in chronically infected human blood. Infect Genet Evol. (2017) 49:300–8. doi: 10.1016/j.meegid.2017.02.006
402. Muñoz-San Martín C, Zulantay I, Saavedra M, Fuentealba C, Muñoz G, Apt W. Discrete typing units of Trypanosoma cruzi detected by real-time PCR in Chilean patients with chronic Chagas cardiomyopathy. Acta Trop. (2018) 185:280–4. doi: 10.1016/j.actatropica.2018.05.004
403. Ramírez JC, Torres C, Curto MLA, Schijman AG. New insights into Trypanosoma cruzi evolution, genotyping and molecular diagnostics from satellite DNA sequence analysis. PloS Negl Trop Dis. (2017) 11:e0006139. doi: 10.1371/journal.pntd.0006139
404. Ramírez JC, Cura CI, da Cruz Moreira O, Lages-Silva E, Juiz N, Velázquez E, et al. Analytical validation of quantitative real-time PCR methods for quantification of trypanosoma cruzi DNA in blood samples from chagas disease patients. J Mol Diagn. (2015) 17:605–15. doi: 10.1016/j.jmoldx.2015.04.010
405. Bontempi IA, Bizai ML, Ortiz S, Manattini S, Fabbro D, Solari A, et al. Simple methodology to directly genotype Trypanosoma cruzi discrete typing units in single and mixed infections from human blood samples. Infect Genet Evol. (2016) 43:123–9. doi: 10.1016/j.meegid.2016.05.026
406. Schijman AG, Bisio M, Orellana L, Sued M, Duffy T, Mejia Jaramillo AM, et al. International study to evaluate PCR methods for detection of Trypanosoma cruzi DNA in blood samples from Chagas disease patients. PloS Negl Trop Dis. (2011) 5:e931. doi: 10.1371/journal.pntd.0000931
407. Rusman F, Tomasini N, Yapur NF, Puebla AF, Ragone PG, Diosque P. Elucidating diversity in the class composition of the minicircle hypervariable region of Trypanosoma cruzi: New perspectives on typing and kDNA inheritance. PloS Negl Trop Dis. (2019) 13:e0007536. doi: 10.1371/journal.pntd.0007536
408. da Cruz Moreira O, Ramirez JC. Genotyping of trypanosoma cruzi from clinical samples by multilocus conventional PCR. Methods Mol Biol. (2019) 1955:227–38. doi: 10.1007/978-1-4939-9148-8_17
409. Sá AR, Dias GB, Kimoto KY, Steindel M, Grisard EC, Toledo MJ, et al. Genotyping of Trypanosoma cruzi DTUs and Trypanosoma rangeli genetic groups in experimentally infected Rhodnius prolixus by PCR-RFLP. Acta Trop. (2016) 156:115–21. doi: 10.1016/j.actatropica.2016.01.006
410. Ortiz S, Zulantay I, Apt W, Saavedra M, Solari A. Transferability of Trypanosoma cruzi from mixed human host infection to Triatoma infestans and from insects to axenic culture. Parasitol Int. (2015) 64:33–6. doi: 10.1016/j.parint.2014.09.005
411. de Oliveira GS, Kawahara R, Rosa-Fernandes L, Mule SN, Avila CC, Teixeira MMG, et al. Development of a Trypanosoma cruzi strain typing assay using MS2 peptide spectral libraries (Tc-STAMS2). PloS Negl Trop Dis. (2018) 12:e0006351. doi: 10.1371/journal.pntd.0006351
412. Lauria-Pires L, Santana JM, Tavares FS, Teixeira AR. Diversity of Trypanosoma cruzi stocks and clones derived from Chagas disease patients: I–Behavioral characterization in vitro. Rev Soc Bras Med Trop. (1997) 30:187–92. doi: 10.1590/s0037-86821997000300003
413. Schenone H, Rojas Mercado A, Castillo D. Estudio comparativo de la sensibilidad y mortalidad de las ninfas III y IV de Triatoma infestans usadas en el xenodiagnóstico de pacientes chagásicos crónicos [Comparative study of sensitivity and mortality of Triatoma infestan nymphs III and IV used in the xenodiagnosis of chronic chagasic patients]. Bol Chil Parasitol. (2000) 55:14–7.
414. Marcet PL, Duffy T, Cardinal MV, Burgos JM, Lauricella MA, Levin MJ, et al. PCR-based screening and lineage identification of Trypanosoma cruzi directly from faecal samples of triatomine bugs from northwestern Argentina. Parasitology. (2006) 132:57–65. doi: 10.1017/S0031182005008772
415. Abolis NG, Araújo SM, Toledo MJ, Fernandez MA, Gomes ML. Trypanosoma cruzi I-III in southern Brazil causing individual and mixed infections in humans, sylvatic reservoirs and triatomines. Acta Trop. (2011) 120:167–72. doi: 10.1016/j.actatropica.2011.08.001
416. Hamilton PB, Lewis MD, Cruickshank C, Gaunt MW, Yeo M, Llewellyn MS, et al. Identification and lineage genotyping of South American trypanosomes using fluorescent fragment length barcoding. Infect Genet Evol. (2011) 11:44–51. doi: 10.1016/j.meegid.2010.10.012
417. Balouz V, Agüero F, Buscaglia CA. Chagas disease diagnostic applications: present knowledge and future steps. Adv Parasitol. (2017) 97:1–45. doi: 10.1016/bs.apar.2016.10.001
418. Wallace A, Sanchez G, Venegas J, Solari A. Lack of cross-reactivity of lytic antibodies with bloodstream forms of Trypanosoma cruzi zymodemes generated in a mouse experimental model. Exp Parasitol. (1995) 80:176–85. doi: 10.1006/expr.1995.1022
419. Zulantay I, Venegas J, Apt W, Solari A, Sanchez G. Lytic antibodies in Trypanosoma cruzi-infected persons with low parasitemia. Am J Trop Med Hyg. (1998) 58:775–9. doi: 10.4269/ajtmh.1998.58.775
420. Krettli AU, Weisz-Carrington P, Nussenzweig RS. Membrane-bound antibodies to bloodstream Trypanosoma cruzi in mice: strain differences in susceptibility to complement-mediated lysis. Clin Exp Immunol. (1979) 37:416–23.
421. McClean MCW, Bhattacharyya T, Mertens P, Murphy N, Gilleman Q, Gustin Y, et al. A lineage-specific rapid diagnostic test (Chagas Sero K-SeT) identifies Brazilian Trypanosoma cruzi II/V/VI reservoir hosts among diverse mammalian orders. PloS One. (2020) 15:e0227828. doi: 10.1371/journal.pone.0227828
422. Di Noia JM, Buscaglia CA, De Marchi CR, Almeida IC, Frasch AC. A Trypanosoma cruzi small surface molecule provides the first immunological evidence that Chagas’ disease is due to a single parasite lineage. J Exp Med. (2002) 195:401–13. doi: 10.1084/jem.20011433
423. Murphy N, Macchiaverna NP, Victoria Cardinal M, Bhattacharyya T, Mertens P, Zeippen N, et al. Lineage-specific rapid diagnostic tests can resolve Trypanosoma cruzi TcII/V/VI ecological and epidemiological associations in the Argentine Chaco. Parasit Vectors. (2019) 12:424. doi: 10.1186/s13071-019-3681-7
424. Martins-Filho OA, Pereira ME, Carvalho JF, Cançado JR, Brener Z. Flow cytometry, a new approach to detect anti-live trypomastigote antibodies and monitor the efficacy of specific treatment in human Chagas’ disease. Clin Diagn Lab Immunol. (1995) 2:569–73. doi: 10.1128/cdli.2.5.569-573.1995
425. Martins-Filho OA, Eloi-Santos SM, Teixeira Carvalho A, Oliveira RC, Rassi A, Luquetti AO, et al. Double-blind study to evaluate flow cytometry analysis of anti-live trypomastigote antibodies for monitoring treatment efficacy in cases of human Chagas’ disease. Clin Diagn Lab Immunol. (2002) 9:1107–13. doi: 10.1128/cdli.9.5.1107-1113.2002
426. Vitelli-Avelar DM, Sathler-Avelar R, Wendling AP, Rocha RD, Teixeira-Carvalho A, Martins NE, et al. Non-conventional flow cytometry approaches to detect anti-Trypanosoma cruzi immunoglobulin G in the clinical laboratory. J Immunol Methods. (2007) 318:102–12. doi: 10.1016/j.jim.2006.10.009
427. Teixeira-Carvalho A, Campos FM, Geiger SM, Rocha RD, de Araújo FF, Vitelli-Avelar DM, et al. FC-TRIPLEX Chagas/Leish IgG1: a multiplexed flow cytometry method for differential serological diagnosis of chagas disease and leishmaniasis. PloS One. (2015) 10:e0122938. doi: 10.1371/journal.pone.0122938
Keywords: Chagas disease, Trypanosoma cruzi, DTU, infectivity, immune response, pathogenesis
Citation: Silvestrini MMA, Alessio GD, Frias BED, Sales Júnior PA, Araújo MSS, Silvestrini CMA, Brito Alvim de Melo GE, Martins-Filho OA, Teixeira-Carvalho A and Martins HR (2024) New insights into Trypanosoma cruzi genetic diversity, and its influence on parasite biology and clinical outcomes. Front. Immunol. 15:1342431. doi: 10.3389/fimmu.2024.1342431
Received: 21 November 2023; Accepted: 26 February 2024;
Published: 09 April 2024.
Edited by:
Annette Dougall, Department of Agriculture, Fisheries and Forestry, AustraliaReviewed by:
Kathryn Marie Jones, Baylor College of Medicine, United StatesAngel Ramos-Ligonio, Universidad Veracruzana, Mexico
Luisa Magalhaes, Universidade Federal de Minas Gerais, Brazil
Copyright © 2024 Silvestrini, Alessio, Frias, Sales Júnior, Araújo, Silvestrini, Brito Alvim de Melo, Martins-Filho, Teixeira-Carvalho and Martins. This is an open-access article distributed under the terms of the Creative Commons Attribution License (CC BY). The use, distribution or reproduction in other forums is permitted, provided the original author(s) and the copyright owner(s) are credited and that the original publication in this journal is cited, in accordance with accepted academic practice. No use, distribution or reproduction is permitted which does not comply with these terms.
*Correspondence: Glaucia Diniz Alessio, glauciabiologia@yahoo.com.br; Helen Rodrigues Martins, helen.martins@ufvjm.edu.br
†These authors have contributed equally to this work and share first authorship
‡These authors share senior authorship