- 1The Second School of Clinical Medicine, Zhejiang Chinese Medical University, Hangzhou, China
- 2Department of General Practice, The Second Affiliated Hospital of Zhejiang Chinese Medical University, Hangzhou, China
Polycystic ovary syndrome (PCOS) is the most common endocrine disorder among women of reproductive age. Although promising strides have been made in the field of PCOS over the past decades, the distinct etiologies of this syndrome are not fully elucidated. Prenatal factors, genetic variation, epigenetic mechanisms, unhealthy lifestyles, and environmental toxins all contribute to the development of this intricate and highly heterogeneous metabolic, endocrine, reproductive, and psychological disorder. Moreover, interactions between androgen excess, insulin resistance, disruption to the hypothalamic–pituitary–ovary (HPO) axis, and obesity only make for a more complex picture. In this review, we investigate and summarize the related molecular mechanisms underlying PCOS pathogenesis from the perspective of the level of signaling pathways, including PI3K/Akt, TGF-β/Smads, Wnt/β-catenin, and Hippo/YAP. Additionally, this review provides an overview of prospective therapies, such as exosome therapy, gene therapy, and drugs based on traditional Chinese medicine (TCM) and natural compounds. By targeting these aberrant pathways, these interventions primarily alleviate inflammation, insulin resistance, androgen excess, and ovarian fibrosis, which are typical symptoms of PCOS. Overall, we hope that this paper will pave the way for better understanding and management of PCOS in the future.
1 Introduction
PCOS is the most common endocrine disorder in women of reproductive age, with an incidence of 6–10% globally, regardless of ethnicity. It is characterized mainly by ovulation dysfunction, hyperandrogenemia (HA), and polycystic ovarian morphology (PCOM). The threshold for PCOM is the presence of more than 20 follicles per ovary and/or an ovarian volume ≥10 ml measured for either ovary via ultrasound (1). According to the Rotterdam criteria, two of the three aforementioned features are required to be present for diagnosis of PCOS. These criteria have been widely endorsed. By contrast, the definition of PCOS from the National Institute of Health (NIH) mainly focuses on two aspects: HA and ovulatory dysfunction (2). Finally, the Androgen Excess Society criteria require the presence of HA along with either ovulatory dysfunction or PCOM, or both. Patients should be carefully evaluated to rule out other conditions that have similar PCOS-like symptoms. Notably, despite the high prevalence of insulin resistance (IR) in PCOS patients, this is not recognized as a diagnostic criterion (3). Based on the three criteria, four different clinical phenotypes are recognized. More recently, two subtypes of PCOS with distinct biochemical characteristics have been identified through phenotypic clustering analysis (4). The first is the reproductive subtype, characterized by higher levels of luteinizing hormone (LH) and sex hormone binding globulin (SHBG), with relatively low BMI and insulin levels. The other subtype is the metabolic subtype, manifesting in the form of higher BMI and insulin levels with relatively low SHBG and LH levels. More active investigation is needed to determine whether these phenotypes reflect the etiology of PCOS and thus can be used to provide tailored treatment for each individual. As PCOS is a multi-factorial and highly heterogeneous endocrine, metabolic, and psychological disorder, its clinical manifestations can be diverse, often leading to delayed diagnosis and misdiagnosis, which can cause long-lasting and distressing complications for patients (Figures 1, 2). Unfortunately, the etiologies of PCOS remain unknown and there is no cure for this condition. In this review, we summarize several key signaling pathways closely involved in the pathogenesis of PCOS: the PI3K/Akt, TLR4/NF-κB, Nrf2/HO-1, AMPK, MAPK, JAK/STAT, Wnt/β-catenin, Notch, Hippo/YAP, TGF-β/Smads, and hedgehog pathways. Additionally, we discuss current therapeutic strategies that target these aberrant signaling pathways.
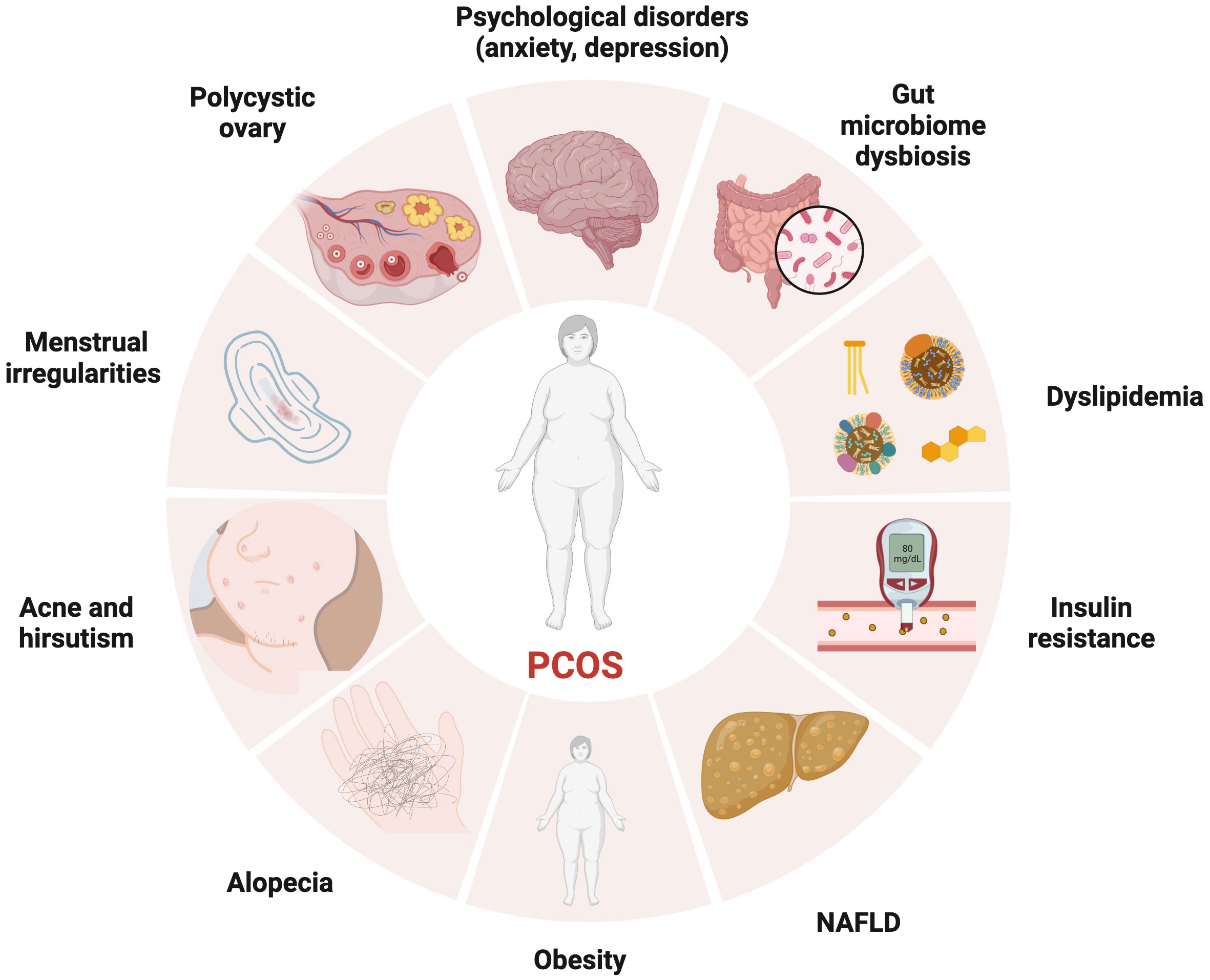
Figure 1 The manifestations of PCOS, a chronic endocrine, metabolic, reproductive, and psychological disorder with various symptoms and signs. It is primarily characterized by ovulation dysfunction (manifesting in the form of menstrual irregularities, such as oligomenorrhea and amenorrhea), hyperandrogenemia (manifesting as hirsutism, acne, or alopecia), and polycystic ovarian morphology.
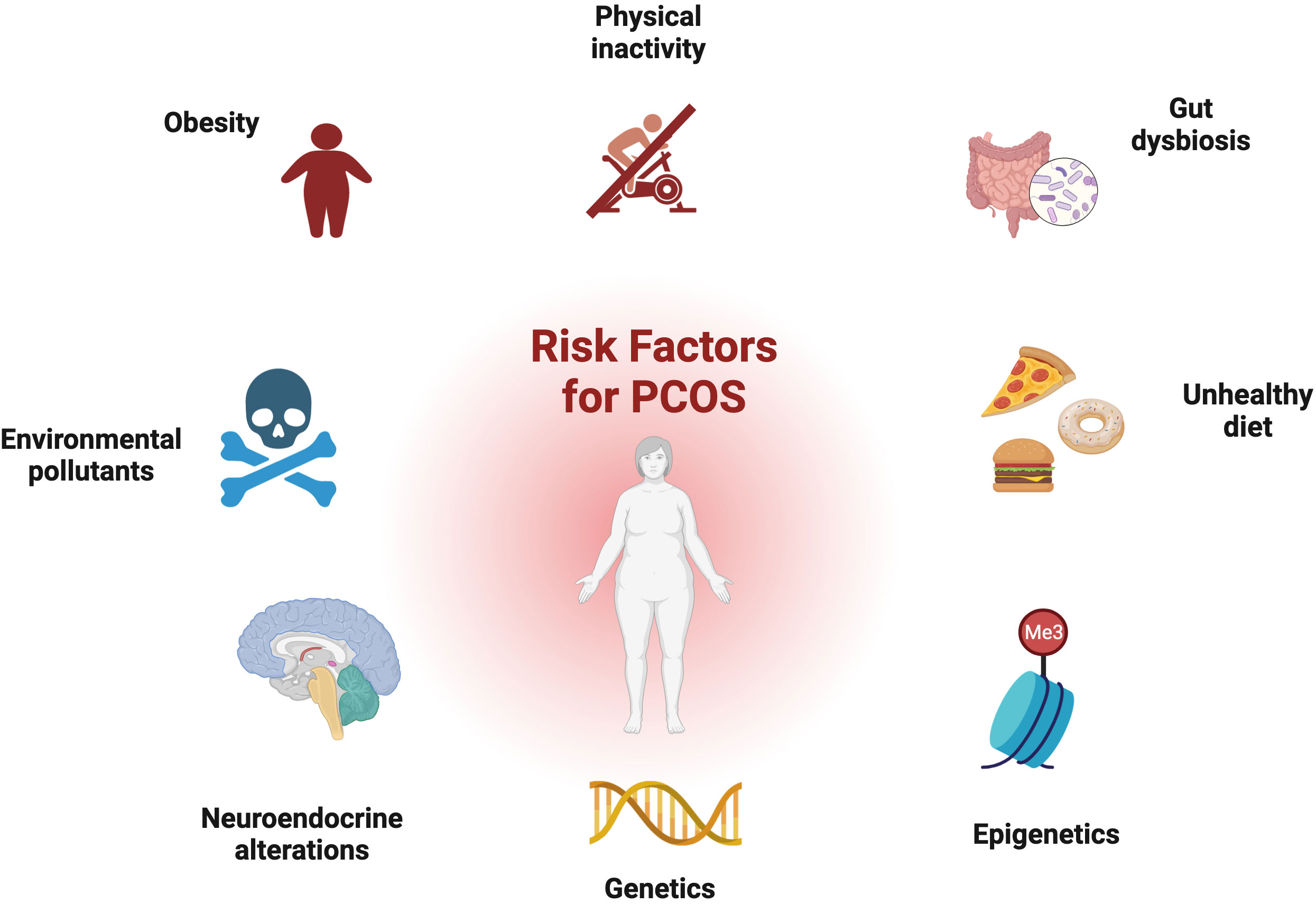
Figure 2 Risk factors for PCOS: genetic and epigenetic factors, disturbance of the hypothalamus–pituitary–ovary (HPO) axis, environmental toxins, dysbiosis of the gut microbiota, and obesity all contribute to development of the disorder.
2 Neuroendocrine imbalance
More recently, attention has been widely paid to the role of the neuroendocrine backdrop in the development of PCOS (5). Simply blocking the androgen receptor (AR) in the brain significantly mitigates PCOS symptoms (6). It has been observed that nearly 70% of PCOS patients present with high levels of LH and an elevated ratio of LH to follicle-stimulating hormone (FSH) as the pulse frequency and amplitude of LH increases. Due to the negative inhibition of estradiol, anti-müllerian hormone (AMH) is secreted by small antral follicles with a maximum diameter of 8 mm. Excess androgen increases the number of pre-antral and small follicles that are growing, resulting in a 2- to 3-fold elevation in circulating levels of AMH in PCOS patients, which further diminishes follicular maturation. High AMH is capable of directly stimulating GnRH neuron activity, favoring LH release and decreasing the sensitivity of granulosa cells to FSH (Figure 3). Collectively, AMH is a dual regulator of follicle growth and hypothalamic GnRH secretion, thus creating a vicious cycle (7). Moreover, hyperandrogenism disrupts the negative feedback from estradiol and progesterone on the gonadal axis. In turn, this leads to the persistent hypersecretion of LH. However, estrogen receptor α, AR, and progesterone receptors are not found in GnRH neurons. Indeed, it has been found that GnRH pulsatility is jointly controlled by upstream regulators, namely, kisspeptin, neurokinin B (NKB), and dynorphin A (DynA), also known as KNDy neurons (5). Okada and colleagues have found that hyperandrogenemia also stimulates the expression of kisspeptin and NKB, whereas it blocks the activity of DynA expression (8). Alongside this study, elevated serum kisspeptin levels in PCOS were observed; these are regarded as a major GnRH pulse generator (5). Theoretically, kisspeptin neurons are located in the anteroventral periventricular nucleus (AVPV) and arcuate nucleus (ARC) in rodents (9). In contrast, kisspeptin neurons are mainly distributed in the infundibular nucleus and the preoptic area (POA) in humans. Kisspeptin stimulates GnRH release and pulsatility by binding to its receptor (KISS1R).
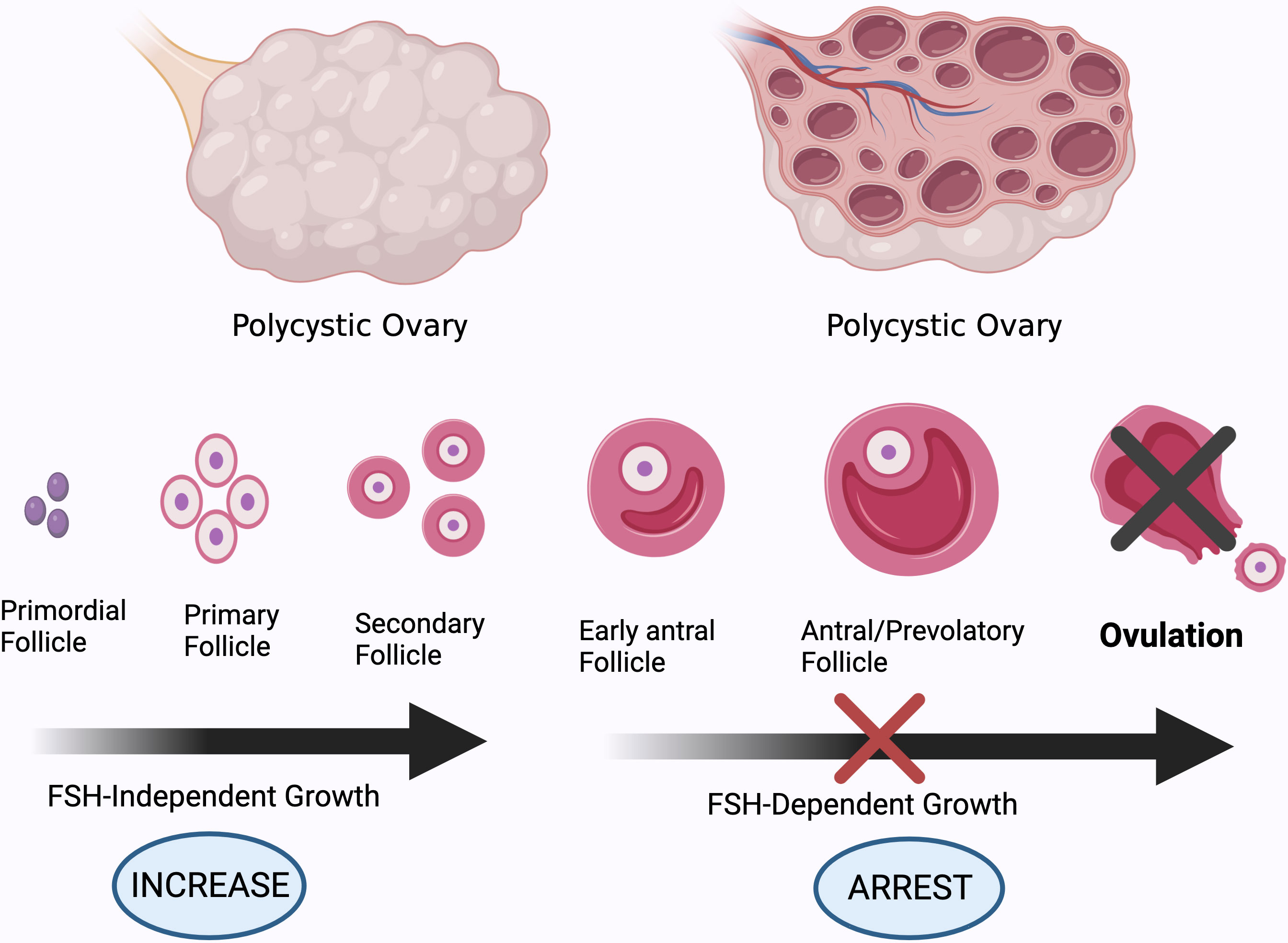
Figure 3 Different stages of development of ovarian follicles. High AMH levels and GnRH pulsatility, and subsequently increased androgen production by theca cells, impair follicle maturation in PCOS patients, resulting in anovulation. Created using BioRender.com.
3 Insulin resistance
Hyperandrogenism promotes the accumulation of subcutaneous fat and the development of insulin resistance by suppressing lipolysis and promoting lipogenesis of adipocytes (10). Obesity may aggravate IR: overall, it promotes insulin secretion while repressing its clearance and degradation (11). Nevertheless, IR occurs in both obese PCOS patients and those of normal weight, regardless of body mass index (BMI). Stepto and his team measured IR in different groups using the hyperinsulinemic–euglycemic clamp technique, which is regarded as the gold standard for evaluation of the beta cell response to insulin. They observed that IR was present in 75% of non-obese PCOS patients, 95% of obese patients, and only 62% of obese healthy controls (12). Hence, it can be deduced that there is an intrinsic defect in insulin receptor signaling in PCOS. Increased serine phosphorylation and reduced tyrosine phosphorylation of insulin receptors and IRS1, leading to impairment of downstream insulin signal transduction, is a primary reason for IR in PCOS (10). Interestingly, one study compared the skeletal insulin sensitivity of lean PCOS patients to their healthy counterparts, and found that there was no defect in the proximal component of insulin signaling. However, the decreased circulating adiponectin levels that impair AMPK activity, as well as the decreased response of pyruvate dehydrogenase (PDH) to insulin stimulation, were found to drive the development of IR in skeletal muscle (13). More recently, a large-scale cross-trait analysis has revealed a genetic link between type 2 diabetes and PCOS, identifying 14 related single-nucleotide polymorphisms (SNPs). Furthermore, these associations are partially independent of BMI (14). Of note, BMI assessment does not accurately reflect the distribution of fat. PCOS patients have more visceral adiposity than controls. Another study found that rs7190396, located near the FTO gene, has the most significant association with PCOS with T2D (15). One genomic study, conducted among genetically homogeneous Italian families with PCOS, identified a number of novel genes associated with PCOS risk, with more studies required for this finding to be replicated in other ethnic groups. Moreover, pathway analysis has indicated that Wnt signaling is the most-affected pathway for risk genes linked to PCOS (16). However, to date, the susceptibility loci identified by genome-wide association studies (GWAS) only explain approximately 10% of the genetic basis of PCOS (17). Overall, these genetic studies indicate that different subtypes of PCOS may share similar underlying biological mechanisms (18).
4 Hyperandrogenism
Patients with PCOS may experience a variety of distressing symptoms caused by excess male sex hormones, such as hirsutism (increased facial or body hair), androgenetic alopecia, and acne. The hyperactive hypothalamic–pituitary–gonadal axis in PCOS patients results in excessive ovarian androgen production in theca cells via upregulation of CYP17A1 enzyme activity (19). Additionally, hyperinsulinemia also reduces hepatic SHBG synthesis, resulting in higher free androgen levels, an active form for the elicitation of biological effects (20). It has been long assumed that ovarian-derived androgen is to blame for the metabolic abnormalities associated with PCOS. Furthermore, previous studies strongly indicate that most steroidogenic enzymes are overexpressed in the theca cells of PCOS patients (21). However, hyperandrogenism can still persist even when ovarian androgen synthesis is suppressed in PCOS patients. In recent years, it has been proposed that adrenal production of 11-oxygenated androgens is the major source of circulating male sex hormones, accounting for a greater proportion than classic androgens (22, 23). This is partly because of the over-reactivity of the adrenal zona reticularis to ACTH stimulation. These findings demonstrate that adrenal hyperandrogenism is also a cause of IR (24). Intriguingly, administration of metformin only lowers testosterone levels, while having no effect on 11-oxygenated androgens (25). Insulin induces androgen production in the adipose tissue in PCOS via upregulation of aldo-keto reductase type 1C3 (AKR1C3) activity, which can convert classical androgen and 11-oxygenated androgens into potent androgen forms, namely, T or DHT and 11-ketotestosterone(11K-T), respectively (26). Obese patients have higher 11-KT levels due to increased AT volume and AKR1C3 activity (22). Moreover, AKR1C3 activates fatty acid synthase (FASN), an enzyme for de novo lipid synthesis, leading to excess lipid accumulation and lipotoxicity (27). To sum up, the ovary, AT, and adrenal glands collectively participate in the hyperandrogenism and IR observed in PCOS patients. Various studies have reported that PCOS patients may face further health implications and poor metabolic status even if their BMI is normal. A correlation has been identified between the prevalence of non-alcoholic fatty liver disease (NAFLD) and hyperandrogenemia, independent of obesity (28). Moreover, women with PCOS with hyperandrogenism have a higher risk of developing T2D in later life than their counterparts with normal androgen levels (29, 30). Another study using data from the UK Biobank revealed sex differences in the effects of testosterone on certain human diseases. A genetically determined increase in testosterone by 1 s.d. increases the risks of polycystic ovary syndrome in women (OR = 1.51; 95% CI: 1.33–1.72), while lowering the risk of type 2 diabetes in men (OR = 0.86; 95% CI: 0.76–0.98) (31). These findings imply that hyperandrogenism is heritable and is not merely a major feature of PCOS; on the contrary, it might be a causative factor of this condition to some extent. Moreover, excess maternal androgen exposure would interfere with placental function and increase the risk of developing PCOS in their female offspring, as well as lowering the quality of sperm in male offspring (32, 33); specifically, this constitutes transgenerational transmission. Due to ethical considerations, animal models of PCOS have been widely established in order to better understand the etiology and pathophysiology of this disease. Dehydroepiandrosterone- (DHEA), letrozole-, and AMH-induced rodent models are frequently used for their highly PCOS-like reproductive and metabolic traits (34, 35). Prenatal androgen exposure helps us to more fully explore the mechanisms of transgenerational transmission, and gene-modified models pave the way for decisive mechanistic studies.
5 Inflammation and oxidative stress
It has been widely accepted that chronic low-grade inflammation is involved in the pathogenesis of PCOS, mainly owing to the excessive fat accumulation observed, particularly in the visceral adipose tissues (36, 37). Hypoxia triggers necrosis of adipose tissues, which later recruits and activates immune defense cells, initiating a complement cascade, and ultimately leading to a pro-inflammatory state. Elevated inflammatory biomarkers include high sensitivity C‐reactive protein (hsCRP), white blood cell counts, cytokines (e.g., IL‐1β, IL‐6, and IL‐18), and chemokines (e.g., MCP‐1 and MIF) (38). Mitochondrial dysfunction, obesity, IR, and unhealthy dietary patterns (for instance, high composition of dietary carbohydrates and high intake of fatty acids) are factors contributing to the development of oxidative stress (OS). OS is the result of an imbalance between oxidants and antioxidants and leads to surplus reactive oxygen species (ROS) (39). The pathological factors mentioned above, as well as hyperandrogenism (40), together play important roles in the development of low-grade inflammation in PCOS, and these factors also interact with one other, thus creating a vicious circle (41, 42). However, it is noteworthy that OS and inflammation are not always detrimental to the female reproductive system. Under some circumstances, they are necessary for ovulation and progesterone synthesis (39).
6 Pathogenic signaling pathways
6.1 The PI3K/Akt signaling pathway
The phosphoinositide-3 kinase/protein kinase B (PI3K/Akt) signaling pathway plays an essential role in cell survival and glucose homeostasis. Based on structural and functional characteristics, there are three classes of PI3K, with class 1 PI3K being the most extensively studied. When insulin is released by β cells, it binds to its receptor. Subsequently, the insulin receptor substrate (IRS) is recruited to insulin receptors, resulting in the activation and phosphorylation of PI3K. Activated class ι PI3K will then transform phosphatidylinositol 4,5-bisphosphate (PIP2) into phosphatidylinositol 3,4,5-trisphosphate (PIP3). As a second messenger, PIP3 binds to the PH domain of AKT in the plasma membrane. Furthermore, with the help of phosphoinositide dependent kinase 1 (PDK1) and mechanistic target of rapamycin kinase complex 2 (mTORC2), respectively, the threonine phosphorylation site (Thr308) and serine phosphorylation site (Ser473) on Akt are phosphorylated (43). Once activated, AKT will exert diverse biological effects downstream as a core part of the PI3K/Akt signaling pathway (44). Theoretically, FSH modulates the expression of IRS2, thus affecting glycogen synthesis and glucose uptake via the PI3K/Akt pathway (45). However, in PCOS patients, elevated LH activity interferes with the expression of FSH-stimulated IRS2. Ultimately, the defective FSH-responsiveness results in glycogen depletion and disruption to follicle growth (46). Hence, any flaw or truncation in insulin signal transduction will impair insulin sensitivity.
6.1.1 The main components and regulators
In muscle or adipose tissue (AT), AKT prompts glucose transporter type 4 (GLUT4) to translocate from the cytoplasm to the cytomembrane, thereby mediating insulin-stimulated glucose uptake. GLUT4 expression in the adipocytes is observed to be reduced in PCOS, and GLUT1 gene expression is not increased in compensation (47). Interestingly, metformin medication is found to have lasting effects in PCOS patients in terms of induction of GLUT4 mRNA expression in AT, even after its withdrawal (48).
6.1.1.1 Forkhead box O transcription factors
FoxOs are a group of direct downstream targets of the PI3K/Akt pathway; when AKT is activated, it will phosphorylate FoxOs and hamper their nuclear translocation, thereby inhibiting the expression of some pro-apoptotic genes. The findings of numerous studies support the view that the FOXO family plays an important role in female reproduction (39). AMH favors autophagy and inhibits activation of FOXO3A, thus preventing premature follicle depletion (49–51). Additionally, one study has demonstrated that FOXO3 expression is increased in non-obese PCOS patients and is related to m6A modification (52). In addition, the level of FoxO1 expression is increased in women with PCOS compared to controls, and this may be related to ovarian inflammation and follicular atresia. Previous studies have found that increased production of pro-inflammatory cytokines is positively correlated with FoxO1 expression in hepatocytes and macrophages (53, 54). Activation of FoxO1 also inhibits the gene expression of SLC2A4 (GLUT4), reducing glucose uptake and causing IR (51). Moreover, angiopoietin-like protein 2 (ANGPTL2) has been shown to be involved in IR in PCOS models via an increase in FoxO1 expression (55).
6.1.1.2 Glycogen synthase kinase-3
Glycogen synthase (GS) is negatively regulated by GSK3, which inhibits glycogen synthesis; in contrast, Akt phosphorylates GSK3 to inhibit its activity. The GSK3 family consists of two isoforms in mammals: GSK3α and GSK3β. Many previous studies have reported that the GSK3β gene is over-activated in PCOS patients (56, 57). Conversely, another study has found that the phosphorylation of GSK3β at the ser9 site is increased in the uterus of rats with PCOS-like symptoms induced with a combination of insulin and human chorionic gonadotropin (hCG). In the same study, Glut7 mRNA was found to be significantly increased and a decline in Glut4 expression was observed in the endometrium in the rat model of PCOS (58).
6.1.1.3 Phosphatase and tension homologue
PTEN acts as a negative regulator: it can convert PIP3 back into PIP2, thereby inhibiting activation of the PI3K/Akt pathway (43). Women with PCOS have been found to exhibit increased endometrial expression of PTEN (59). Ubiquitin-specific protease 25 (USP25) is a deubiquitinating enzyme that prevents degradation of PTEN. Notably, USP25 expression is found to be increased in PCOS patients, thus stimulating excessive PTEN synthesis (60). Serum amyloid A1 (SAA1), an acute-phase protein produced in response to inflammation, infection, and trauma, has also been found to be increased in PCOS patients. Its concentration in follicular fluid is 10 times higher than that in blood circulation. Researchers have found that this can induce the PTEN expression and, as a result, suppress downstream signaling (61).
6.1.1.4 Lymphocyte adaptor protein
Many studies have confirmed that LNK (SH2B3) is a regulatory factor in insulin signaling via its effect on glucose uptake. LNK knockdown in mice results in increased GLUT4 activity in adipose tissue (62). LNK expression is significantly higher in women with PCOS with IR compared to patients without IR and control groups, and LNK suppresses activation of the insulin-meditated PI3K pathway (63). It has also been found to promote granulosa cell apoptosis via the AKT/FOXO3 pathway in PCOS patients (64).
6.1.2 Therapeutic strategies
6.1.2.1 Gene therapy
MicroRNAs (miRNAs) are a large family of small non-coding RNA sequences that negatively regulate gene expression post-transcriptionally (65). They are linked to many diseases, including PCOS. The follicular fluid-derived exosomal miR-18b-5p, targeting PTEN, might be considered as a potential therapy for reducing IR in PCOS (66). Additionally, miR-29c-3p improves glucose metabolism via inhibition of FOXO3 translocation (67).
6.1.2.2 Traditional Chinese medicine
Several studies imply that berberine is safe and has a beneficial effect in treatment of PCOS, targeting multiple pathways, such as by decreasing the synthesis of excessive androgen, facilitating the expression of GLUT4 proteins, and alleviating IR (68, 69). It has also been found that certain Chinese decoctions, such as Heqi San, Liuwei Dihuang pills, and Guizhi Fuling Wan, have relevant physiological effects, improving insulin resistance and ameliorating sex hormone disturbances in rat models through activation of the PI3K/AKT pathway (70–72).
6.1.2.3 Melatonin
MT is a neuro-hormone that is primarily synthesized and secreted by the pineal gland and is essential for circadian rhythm. As a multifunctional molecule, it also participates in the regulation of reproductive functions via multiple targets. An increasing body of literature strongly indicates that disruption to the circadian rhythm is closely linked to the progression of PCOS (73). Studies have revealed that long-term exposure to light results in increased levels of FSH and estradiol. Consistent with this, prolonged darkness has also been found to lead to hyperandrogenism in PCOS via downregulation of melatonin receptor 1A (74). The concentration of MT in follicular fluid (FF) has been found to be significantly lower in women with PCOS than in healthy controls. Interestingly, this is associated with increased levels of inflammatory markers (75). MT treatment effectively attenuates mitochondrial injury and oxidative stress in granulosa cells (GCs), functioning as a SIRT1 activator to activate the PDK1/Akt pathway (76, 77). Additionally, administration of MT may improve oocyte development by increasing the expression of oocyte maturation-related genes, namely, growth differentiation factor 9 (GDF9) and bone morphogenetic protein 15 (BMP15) (78); it also reverses the low progesterone levels observed in PCOS by upregulating StAR expression in GCs (79). MT is also capable of increasing FSH secretion via stimulation of the pituitary gland (80). Overall, MT protects ovarian functions by mitigating inflammation, cellular apoptosis, and OS; these effects are primarily or partially dependent on activation of the PI3K/AKT pathway.
6.2 The TLR4/NFκB signaling pathway
Toll-like receptor 4 (TLR4)/nuclear factor-κB (NF-κB) signaling has been extensively studied and is a well-established signaling pathway mediating inflammatory responses. Working as a pattern recognition receptor (PRR), TLR4, unlike other members of the toll-like receptor (TLR) family, can engage all four kinds of toll-interleukin receptor (TIR) domain-containing adaptor proteins: MyD88, TIRAP, TRAM, and TRIF (81). In general, with the assistance of co-receptors CD14 and MD2, TLR4 is activated by pathogen-associated molecular patterns (PAMPs), such as LPS, and damage-associated molecular patterns (DAMPs), e.g., oxLDL and saturated fatty acid; TIRAP-MyD88 adaptors are subsequently recruited to TIR domains. Eventually, the downstream IKK complex is activated, causing the IκB protein to be degraded; NF-κB subsequently enters the nucleus, further initiating the expression of a series of inflammatory molecules, such as TNF-α, IL-1, and IL-18 (82, 83). One study has indicated that over-expression of MIF stimulates hyperactivity in the NF-κ pathway in a DHEA-induced PCOS model, whereas the application of MIF antibody is associated with significant recovery from PCOS symptoms (38). Hu et al. have demonstrated that endometrial inflammation in PCOS patients is induced by TLR4/IRF-7/NFκB signaling (84). High levels of TNF-α may repress endometrial GLUT-4 expression in PCOS via NFκB activation, resulting in endometrium dysfunction (85, 86). Additionally, elevated inflammatory factors are observed in both obese patients and those of normal weight, with higher levels in the obese group (87), suggesting that obesity may exacerbate inflammatory states (88). This chronic inflammation is thought to be systemic rather than regional, with inflammatory markers travelling through the bloodstream to the ovaries, altering the micro-environment of the follicular fluid, thereby triggering the inflammatory cascade and leading to dysfunctional and aberrant GCs (89). Furthermore, miR-93-5p has also been found to contribute to the progression of apoptosis and ferroptosis in GCs in PCOS via NF-κB signaling (90).
6.2.1 Inflammation and insulin resistance
High-mobility group box 1 (HMGB1) is a multi-functional small nuclear protein regulating gene transcription (91). It functions as a DAMP for activation of TLR4 signaling, with the help of MD-2, when it is released into the extracellular matrix (92). HMGB1 is required in order for LPS and nucleic acids to manifest their full toxicity (93). Circulating HMGB1 has been found to be increased in PCOS. HMGB1 has been linked to inflammation, impaired insulin sensitivity, and endothelial dysfunction in PCOS patients (94, 95). At the transcriptional level, HMGB1 is also the direct mRNA target of miR-129 (96), while lncRNA ZFAS1 could competitively bind to miR-129 to induce HMGB1 expression. Moreover, the abundance of miR-155 in the FF in PCOS is negatively correlated with HMGB1 concentration (97). The enhanced autophagy induced by increased HMGB1 content may be a contributing factor in IR in the GCs of PCOS patients, embodied by over-expression of ATG7 and a decrease in p62 levels (98). The release of HMGB1 must undergo acetylation and nuclear-to-cytoplasmic translocation processes (99). Thus, increasing the activity of Sirtuin1 (SIRT1), an NAD+ dependent deacetylase, can prevent HMGB1 from being released into extracellular space, thereby reducing the inflammatory response (100). Hence, the alleviation of the metabolic disorders seen in PCOS by treatment regimens such as melatonin, resveratrol, and metformin is at least partially mediated by the activation of SIRT1 (77, 101, 102). After treatment with myo-inositol (MYO) in combination with alpha-lipoic acid (ALA), levels of HMGB1 in adolescents with PCOS have been found to return to normal (25, 103). Cryptotanshinone (CRY) also exerts its therapeutic efficacy via HMGB1/TLR4/NF-κB signaling (104).
6.2.2 The inflammasome and exosomes
The inflammatory cytokines L-1β and IL-18 are found to be increased in PCOS patients; these are related to ovulation, and moreover, the structure of IL‐18 is similar to that of the IL‐1 family. Maturation of these two inflammatory cytokines occurs by means of the activation of inflammasomes (105). Inflammasomes are multiple-protein complexes that are assembled and initiate inflammation in response to harmful stimuli. Dysregulation of inflammasomes is closely linked to numerous human diseases, including neurodegenerative diseases, cancers, and vascular diseases. There are two categories of inflammasomes: canonical and non-canonical inflammasomes. Generally speaking, the canonical inflammasomes are comprised of three components: sensor proteins (such as NLRP1, NLRP3, NLRP6, NAIP/NLRC4, AIM2, and PYRIN), the adaptor ASC, and effectors (pro-caspase1). When DAMPs or PAMPs are recognized by the PRRs, ASC and pro-caspase-1 are recruited to the complex. Subsequently, pro-caspase-1 is activated to form caspase-1, and pro–IL-1β, pro–IL-18, and gasdermin D are then cleaved into active forms (106). Among these, NLRP3 and AIM2 inflammasomes have been detected in PCOS patients; their presence may be driven by hyperandrogenism and fatty acids (107–109). Acetate, a histone deacetylase inhibitor, can suppress the activation of the NLRP3 inflammasome and restore the overactive kisspeptin system in rat models (110). MiR-1224-5p may inhibit activation of the NLRP3 inflammasome by targeting FOXO1 (111). Pioglitazone and metformin dual therapy could mitigate the psychological distress of PCOS patients by reducing NLRP3 inflammasome activation (112, 113), while plumbagin has been found to reduce pyroptosis of GCs. The latter inhibits the activity of WTAP, a key regulator of the RNA N6-methylase complex, consequently destabilizing the ASC mRNA and inhibiting activation of the NLRP3 inflammasome (114).
Exosomes are cell-created extracellular vesicles with sizes in the range of 50–150nm, containing various bioactive molecules. They can be transferred from one cell to another, thus enabling signal transduction and intercellular communication. In recent years, researchers have identified the dual regulatory role played by exosomes, which can negatively or positively affect the activation of inflammasomes. The function of an exosome is dependent on which cargos it carries and where it originates (115). The S100‐A9 protein in exosomes from the follicle fluids of PCOS patients may activate the NF‐κB signaling pathway (116), while human umbilical cord mesenchymal-stem-cell-derived exosomes (hUC-MSC-exos) can inhibit NF-κB signaling via stimulation of the phosphorylation and degradation of Ik-B, increasing the expression of anti-inflammatory cytokines IL-10, and are capable of activating M2 macrophage polarization. As a consequence, inflammation in the ovary is ameliorated (117). Exosomal miR-323-3p derived from adipose mesenchymal stem cells (AMSCs) can protect cumulus cells from apoptosis via direct inhibition of the over-expression of PDCD4 (PMID: 31549864). Moreover, it has been reported that the miR-21-5p carried by AMSC-EXOs can migrate to the liver and activate the IRS1/AKT pathway, thereby mitigating hepatic IR (118). To summarize, these findings indicate that exosomes derived from stem cells can inhibit inflammation through a variety of mechanisms, with microRNAs in stem cells serving as the key players (119, 120). Additionally, hsa-miR-1299, hsa-miR-6818-5p, hsa-miR-192-5p, and hsa-miR-145-5p are highly expressed in the blood of PCOS patients; therefore, these exosomal miRNAs might be promising biomarkers for the early diagnosis of PCOS (121, 122).
6.2.3 The gut microbiota and inflammation
Accumulating evidence has demonstrated the close relationship between the gut microbiome and PCOS. Various studies have indicated that the composition of the gut microbiota is altered in PCOS patients, with reduced alpha and beta diversity and decreased Lactobacilli, Bifidobacteria, and Prevotellaceae content, while the abundance of Bacteroides vulgatus is increased (123, 124). This disturbance of the intestinal flora disrupts the integrity of the gut barrier, increasing gut permeability to LPS, and the resultant activation of the TLR-4/NF-κB-mediated inflammatory response. Moreover, these changes are also influenced by HA. It has been proposed that prenatal androgen exposure could lead to dysbiosis of the gut microbiota. One study has revealed an inverse correlation between alpha diversity and circulating testosterone levels (125).
6.2.4 Therapeutic strategies
Supplementation with α-Linolenic acid extracted from flaxseed oil has exhibited strong potential in mitigating PCOS-related symptoms: it significantly reduces the levels of LPS and inflammatory factors, alters microbiota composition, and increases the abundance of short-chain fatty acids (125). Additionally, it has been indicated that regimens such as the Bu Shen Hua Zhuo formula and the Shaoyao-Gancao decoction relieve the symptoms of rats with PCOS by modifying the gut microbiota to suppress inflammation (126, 127). Furthermore, IL-22 links intestinal bacteria to IR and androgen excess. Serum IL-22 concentrations are significantly lower in PCOS individuals. In terms of mechanism, increased levels of Bacteroides vulgatus decrease the levels of conjugated bile acids. This results in downregulation of intestinal type 3 innate lymphoid cells (ILC3s) to produce IL-22 in a GATA3-dependent manner (128). However, treatment to increase IL-22 inhibits the release of inflammatory cytokines CCL2, CCL20, and IL-1β, ultimately improving ovarian function (124).
6.2.4.1 Nutritional supplements
Some edible supplements, including coenzyme Q10, fish oil, omega-3 fatty acids, and vitamin E, have shown effectiveness in suppressing the gene expression of some inflammatory mediators, including IL-1, IL-8, and TNF-α, in women with PCOS (129–131).
6.3 The Keap1/Nrf2 signaling pathway
The Kelch-like ECH-associated protein 1 (Keap1)/nuclear factor erythroid 2-related factor 2 (Nrf2) system functions as a primary antioxidative defense in humans. Keap1 works as a sensor for ROS or electrophiles, whereas Nrf2 acts as a transcriptional factor that regulates the bulk of antioxidant gene expression. Under normal conditions, Nrf2 binds to the KEAP1-RBX1-CUL3 E3 ubiquitin ligase complex, and is then degraded by the ubiquitin–proteasome system in the cytoplasm. Additionally, the activity of Nrf2 is also regulated by p62, a substrate of autophagy, via the inactivation of Keap1 (132). However, in a stressed state, the structure of Keap1 changes, thus preventing Nrf2 degradation. Subsequently, the newly synthesized Nrf2 translocates to the nucleus, where it heterodimerizes with sMAF proteins. The heterodimer then recognizes AREs/EpREs, leading to the activation of downstream Nrf2-mediated genes, promoting the synthesis of certain antioxidant proteins or detoxification enzymes, such as heme oxygenase-1 (HO-1) and NADPH quinone oxidoreductase-1 (NQO-1). HO-1 is a member of the heme oxygenase family, encoded by the HMOX1 gene. As a rate-limiting enzyme, HO-1 catalyzes heme into biliverdin, carbon monoxide (CO), and free iron (Fe2+); biliverdin can then be converted into bilirubin. These metabolic molecules are thought to be potent antioxidants. Serum levels of HO-1 are considerably lower in non-obese PCOS patients, due to its exhaustion (78). One study has demonstrated that the miR-873-5p inhibitor can reduce ROS by directly targeting HO-1 (133). Melatonin supplementation also improves the poor quality of oocytes by suppressing nitric oxide (NO) synthetase and NO release, upregulating Nrf2 and downstream HO-1 (78). Humanin is a mitochondria-derived peptide. It has been reported that supplementing with humanin analogue (exogenous humanin) improves both IR and OS in PCOS patients via the Keap1/Nrf2 and PI3K/Akt pathways (134, 135).
6.3.1 Crosstalk between NF-κB and the Keap1/Nrf2 pathway
Many previous studies have demonstrated the complex interactions between the keap1/Nrf2 and NF-κB pathways in anti-inflammatory and proinflammatory responses (136). First, Nrf2 activation may inhibit the release of certain inflammatory cytokines, cell adhesion molecules, and matrix metalloproteinases (MMPs). As a result, NF-κB activation is suppressed. Additionally, Nrf2 may disrupt the NF-κB nuclear translocation process (137). On the transcriptional level, NF-κB competes with Nrf2 for the binding site of CBP, a co-activator of Nrf2. Additionally, p65 may recruit the co-repressor HDAC3, decreasing the expression of downstream ARE-driven genes. Moreover, Nrf2 may interfere with the assembly of the NLRP3 inflammasome. Therefore, Nrf2 inducers have strong potential for use in anti-inflammatory therapeutic strategies in PCOS (Figure 4).
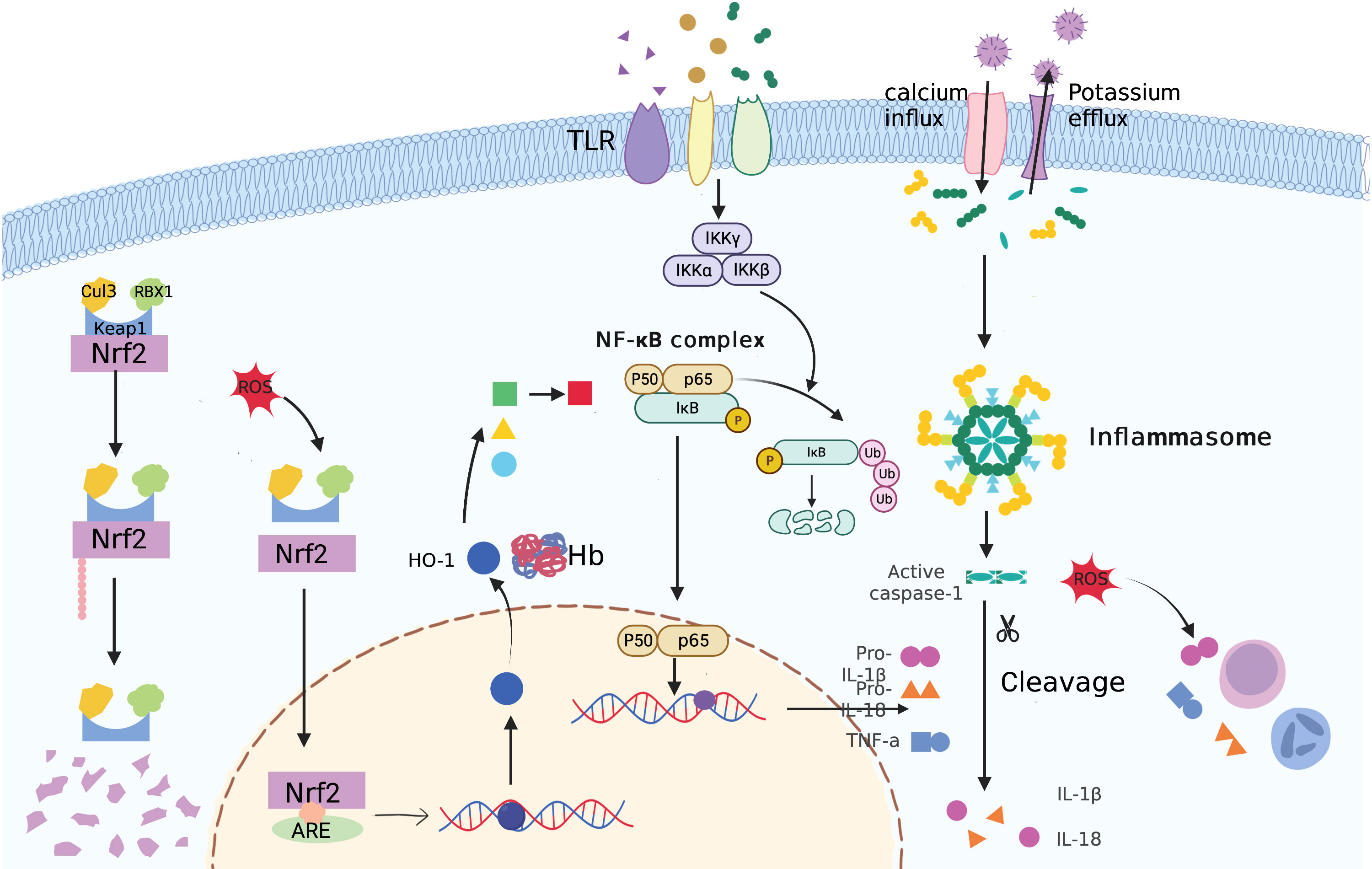
Figure 4 An illustration of inflammasome, TLR4/NFκB, and Keap1/Nrf2 signaling pathways in PCOS pathogenesis.
6.3.2 Crosstalk between AMPK and the Keap1/Nrf2 pathway
It has been demonstrated that, as a upstream modulator, AMPK activates NRF2 by promoting Nrf2 phosphorylation at serines 374, 408, and 433 (138). However, the interesting aspect of this is that, when AMPK kinase is inhibited by compound C, the basal level of Nrf2 is unaffected (139). AMPK may also regulate Nrf2 activity via an indirect pathway: it may deactivate GSK3β via PTEN–PI3K–AKT–GSK-3β cascade, thereby promoting Nrf2 ubiquitylation and degradation. Sulforaphane (SFN), considered to be a potent Nrf2 inducer, has extensive presence in certain cruciferous vegetables, including broccoli and kale (140). Numerous studies have proven its anti-aging, anticancer, and antioxidant properties via various mechanisms (141, 142). In the ovary, SFN reduces tumor invasion and angiogenesis through inhibition of the HIF-1 pathway (143). SFN has also been shown to exert a protective effect on GCs in PCOS patients via activation of the AMPK/Nrf2 pathway (144). Additionally, Ji and colleagues found that salidroside may reduce ROS production and apoptosis of GCs in PCOS patients through activation of AMPK/Nrf2 signaling (145).
6.3.3 Nicotinamide adenine dinucleotide phosphate oxidase 4
NOX4 belongs to the nicotinamide adenine dinucleotide phosphate (NADPH) oxidase (NOX) family, which is a primary participant in endogenous ROS production (146). One publication has reported the involvement of NOX4 in inducing ROS production and impairing skeletal insulin sensitivity in testosterone-induced models of PCOS (147). Researchers have also demonstrated that NOX and Keap1/Nrf2 play interacting roles in the control of ROS levels. NOX4 deficiency has been shown to ease the symptoms of rats with PCOS (148). Intriguingly, over-expression of NOX4 may in turn activate expression of Nrf2, thus exerting a protective effect on cardiomyocytes under chronic conditions of overload (149). However, similar phenomena have not been observed in the case of lung or kidney injuries (150).
6.4 The AMPK pathway
Adenosine monophosphate (AMP)-activated protein kinase (AMPK), which is present in almost all eukaryotes, is a highly conserved heterotrimeric serine/threonine protein kinase that regulates energy metabolism (151). It consists of α, β, and γ subunits, among which α has α1 and α2 subunits, β has β1 and β2 subunits, and γ has γ1, γ2, and γ3 subunits. When there is an energy deficiency, the ratio of AMP to ATP rises and AMPK is activated to produce more or consume less ATP in order to maintain energy balance (152). Reduced expression of α1AMPK is observed in the GCs of women with PCOS, and this is accompanied by higher steroidogenesis due to the increased activity of 3β-hydroxysteroid dehydrogenase (3βHSD) and P450 side-chain cleavage enzyme (P450scc). With the deletion of α1AMPK in rodent models, antral follicle numbers are consistently higher, along with a reduction in the population of pre-ovulatory follicles (153). Glycolysis is found to be enhanced in the GCs of PCOS patients. This increased activity of glycolysis is a marker of activated mTOR signaling and inactivation of AMPK, resulting in the excessive activation of primordial follicles and a reduction in resting follicle storage (154). Another study has found that the skeletal IR of PCOS patients of normal weight is not related to proximal defects of the insulin signaling pathway, but is instead associated with circulating adiponectin levels and subsequent inhibition of AMPK kinase (13, 155). Of note, more recent research has found that AMPK activity is suppressed in subcutaneous adipose tissue (SAT), while insulin sensitivity is preserved in visceral adipose tissue (VAT) by upregulation of AMPK via overfeeding in rat models of PCOS (156). Hence, the theory of limited expandability of SAT has been proposed: namely, that ectopic fat deposition in the muscle and liver and IR are followed by excessive fat accumulation in SAT (157).
6.4.1 Crosstalk between AMPK and SIRTs
SIRTs are a family of NAD+-dependent histone deacetylases that regulate a variety of physiological functions. To date, seven members of the family (SIRT1–SIRT7) have been identified. SIRT1 can deacetylate LKB1, an upstream regulator of AMPK, increasing the cytoplasmic localization of LKB1 and thus promoting AMPK activation. In turn, AMPK enhances SIRT1 activity by boosting cellular NAD+ levels. Loss of SIRT1 leads to a larger population of early-stage follicles and to ovulation (158). A number of reports have demonstrated that increasing SIRT1 activity can alleviate PCOS (159). Additionally, SIRT1 helps with scavenging of advanced glycation end products (AGEs) through upregulation of glyoxalases, a key component of the anti-glycation defense system (160). As a mitochondrial protein, SIRT3 is found to be decreased in PCOS, and this deficiency contributes to the over-production of ROS and decreased mitochondrial membrane potential (161). Proliferator-activated receptor gamma coactivator-1alpha (PGC-1alpha) acts on the murine SIRT3 promoter; the resulting increase in expression of SIRT3 protects the mitochondria from OS damage (162). Interestingly, SIRT3 levels are also elevated in DHT-induced models of PCOS, supporting the concept of adaptive response (160). The increased expression of SIRT1 and SIRT3 induced by low-level oxidative stress may be the result of a compensatory defense, thus favoring autophagy, which is characterized by increased levels of autophagy marker LC3-II and decreased P62 levels in GCs; consequently, the effect is to promote ovarian health. Studies have shown that androgen can induce autophagy in the GCs in PCOS in a dose-dependent pattern; elevated serum homocysteinem levels are also involved (163, 164). Notably, a harsh environment might even lead to degradation of proteasome-mediated SIRTs and inhibition of autophagy.
6.4.2 Therapeutic strategies
In theory, restoration of AMPK expression is expected to be a potential therapeutic strategy.
6.4.2.1 Drugs
Metformin is a well-known AMPK activator: it phosphorylates AMPK at the T172 site, thus blocking the expression of matrix metalloproteinase-2 (MMP2) and MMP-9, which may be related to abnormal follicular atresia (165, 166). A glucagon-like peptide 1 (GLP-1) receptor agonist can also be prescribed in order to lower glucose levels. Exenatide and liraglutide have both exhibited beneficial effects in PCOS patients when used alone or in combination with metformin, working via the AMPK/SIRT1 pathway (102, 167). Myo-inositol (MYO) plays a role in improving insulin sensitivity by activating AMPK and increasing GLUT-4 levels. Previous clinical trials have demonstrated that MYO treatment improves outcomes for PCOS patients who are receiving treatment with assisted reproductive technology (168, 169). Moreover, calcitriol supplementation elicits a cardioprotective effect by blunting PCOS-related cardiac remodeling (170). Considering the decreased NAD+ levels occurring in the GCs of PCOS patients, nicotinamide (NAM) and its metabolite N1-Methylnicotinamide (MNAM) have been shown to have therapeutic potential in PCOS (171, 172). In terms of the mechanism, low-dose MNAM supplementation triggers transient ROS generation induced by increased aldehyde oxidase 1 (AOX1) activity. In addition, these agents alleviate hyperandrogenism through inhibition of AMPK-activation-mediated expression of CYP17A1 (173).
6.4.2.2 Phytochemicals
Quercetin (QUR), a natural phytochemical with a high flavonol content, can help to ameliorate metabolic and endocrine abnormalities in PCOS, such as by improving IR, inflammation, and hyperandrogenism (174). Upon treatment with QUR, activity of AMPK and SIRT1 is increased, reducing fat accumulation by modulating the secretion of adipocytokines (158, 175). Other compounds, including fisetin and baicalin, have both exhibited beneficial effects via targeting of AMPK (176, 177).
6.5 The MAPK pathway
The mitogen-activated protein kinase (MAPK) signaling cascades are composed of three main kinases: MAPK kinase kinase kinase (MAPKKK), MAPK kinase kinase (MAPKK), and MAPK. These transmit external signals to downstream effectors step by step to regulate a number of critical biological processes (178). Three classic MAPK signaling pathways are extracellular signal-regulated kinase (ERK), p38 MAPK, and c-Jun N-terminal kinase (JNK). ERK is mainly activated by stimuli such as growth factors and mitogens, whereas JNK and p38 are often activated by stress and inflammatory mediators (179). One study has identified a possible role of IL-15 in the development of PCOS: the increased IL-15 level is derived from GCs and induces the phosphorylation of p38 MAPK and JNK, promoting excessive androgen synthesis (180). It has been verified that plasma leptin may be a reliable predictor of PCOS, and high leptin levels are strongly associated with both hyperandrogenism and hyperinsulinemia (181). Additionally, high leptin concentrations may cause oocyte dysfunction (182). Decreased expression of Sam68, an RNA binding protein, links the elevated serum leptin levels to HA. Mechanically, downregulation of Sam68 blocks the aromatase enzyme in response to leptin, thus causing leptin resistance (183), while over-expression of Sam68 restores insulin sensitivity by modulating IRS-1 expression to activate the PI3K and MAPK pathways (184). These results illustrate the dual regulatory functions fulfilled by Sam68 in endocrine dysfunction among PCOS patients. Increased p62 levels in the theca cells in PCOS, representing inhibition of autophagy, cause oxidative damage and hyperandrogenism via activation of the p38 and JNK pathways (185). It is of interest that autophagy is enhanced in GCs and causes GC apoptosis. Under DHT stimulation, the promoters of Map3k1 and Map1lc3a are hypomethylated, leading to increased expression of autophagic-related proteins. This finding implies that MAPK/p53 pathway activation of GCs is driven by dihydrotestosterone (DHT) at the transcriptional level (186). As a result of its binding to the EGFR, elevated levels of heparin-binding epidermal growth factor-like growth factor (HB-EGF) in the FF of PCOS patients activate the cAMP-PKA-dependent JNK and ERK1/2 pathways. Consequently, FOXO1 promotes Ca2+ influx and initiates excessive estrogen synthesis, ultimately leading to estrogen-induced mitochondrial dysfunction and apoptosis of GCs (187). However, other studies have revealed inhibition of the ERK pathway and reduced HB-EGF levels in PCOS patients (188). Expression of StAR and subsequently increased progesterone synthesis could be modulated by upregulation of ERK activity (189, 190). A series of studies have demonstrated that administration of an NK3R antagonist (fezolinetant) leads to a reduction in LH secretion and attenuates symptoms of PCOS by reducing GnRH pulse generation (191). Of note, a recent study has indicated that NKB and NK3R genes are also highly expressed in the ovary, over-activating the MAPK-ERK pathway and disrupting oxidative metabolism (192, 193).
6.5.1 Therapeutic strategies
6.5.1.1 Drugs
The medication metformin has also been found to alleviate ER triggered by hyperandrogenism via repressing the p38MAPK signaling (194). It has been reported that a 400 mg/kg dose of berberine significantly improves insulin sensitivity in rats by increasing GLUT4 expression via inactivation of MAPK and activation of PI3K/AKT signaling (69).
Cangfudaotan decoction exerts an anti-apoptotic effect and restores mitochondrial functions via modulation of the phosphorylation of apoptosis signal-regulating kinase 1 (ASK1) protein; this suppresses downstream JNK activation, thus upregulating Bcl-2 and Bax and cleaving caspase-9 and -3 to limit apoptosis (195).
6.5.1.2 Brown adipose tissue transplantation
BAT activity is dysregulated in PCOS, with an increase in white adipose tissue in its place. Excess androgens inhibit the thermogenesis of BAT and mitochondrial biogenesis, with a reduction in the thermogenic markers uncoupling protein type 1 (UCP1) and proliferator-activated receptor gamma coactivator-1 alpha (PCG-1) (196). However, BAT transplantation from healthy recipients with ample bioactive compounds, such as adipocytokine, could restore BAT function. KEGG pathway analysis has revealed that the MAPK, PI3K and Wnt pathways are involved in this process (197, 198).
6.5.1.3 Gene therapy
miR-14 has been found to exert a protective role in GCs in PCOS patients via inhibition of p38 MAPK and ERK signaling (199).
6.6 The JAK/STAT pathway
The Janus kinase (JAK)/signal transducer and activator of transcription (STAT) pathway is crucial for cell proliferation, differentiation, and apoptosis. The JAK family consists of four members, namely, JAK1, JAK2, JAK3, and TYK2, and there are seven types of STATs (200).
When a cytokine binds to its corresponding receptor, the receptor-associated JAK is activated. This then stimulates the phosphorylation and dimerization of the STAT. Subsequently, the activated STAT dimer translocates to the nucleus, where it regulates the expression of target genes (201). In the bovine ovary, STAT4 is regarded as a transcription regulator in follicular growth (202). A polygenic integrative analysis has provided evidence that STAT function is significantly dysregulated in the ovary in PCOS (203). It has also been confirmed that the levels of phosphorylated STAT3 are elevated in the placentas of PCOS patients (204). The elevated IL-6 levels observed in PCOS, particularly in the obese phenotype, might be a possible explanation. Over-expression of IL-6 and IL-11 stimulates the proliferation of adipocytes in rat adipose tissues, which is followed by activation of the STAT3 pathway (205). In trans IL-6 activation, the highly expressed IL-6 firstly binds to its soluble receptor (sIL-6R); the complex later binds to gp130 molecules, thus mediating downstream JAK/STAT3 activation and exerting its pro-inflammatory nature in PCOS patients. The classical signaling pathway, in contrast, is anti-inflammatory and is initiated by IL-6 binding to membrane-bound IL-6R with the aid of gp130 (206).
6.6.1 Therapeutic strategies
miR-520h has been found to directly target IL-6R, thereby inhibiting the development of PCOS (207). Tofacitinib, one type of JAK inhibitor, was initially developed for the treatment of autoimmune diseases, such as rheumatoid arthritis (RA) (208). It is of interest that inhibition of miR-199a-5p reduces apoptosis in GCs of PCOS patients via activation of JAK/STAT3 pathway. However, this protective effect is counteracted by treatment with tofacitinib (209). Moreover, the flavonoids extracted from Nervilia fordii have shown potent effectiveness in reversing PCOS-related traits via regulation of IL-6 through the JAK2/STAT3 pathway (210). A troxerutin regimen is reported to decrease IR through activation of JAK1/STAT3 signaling in beta cells (211); this decreases the abundance of Bifidobacterium and alters the bile acid profile, ultimately boosting serum IL-22 levels (Figure 5).
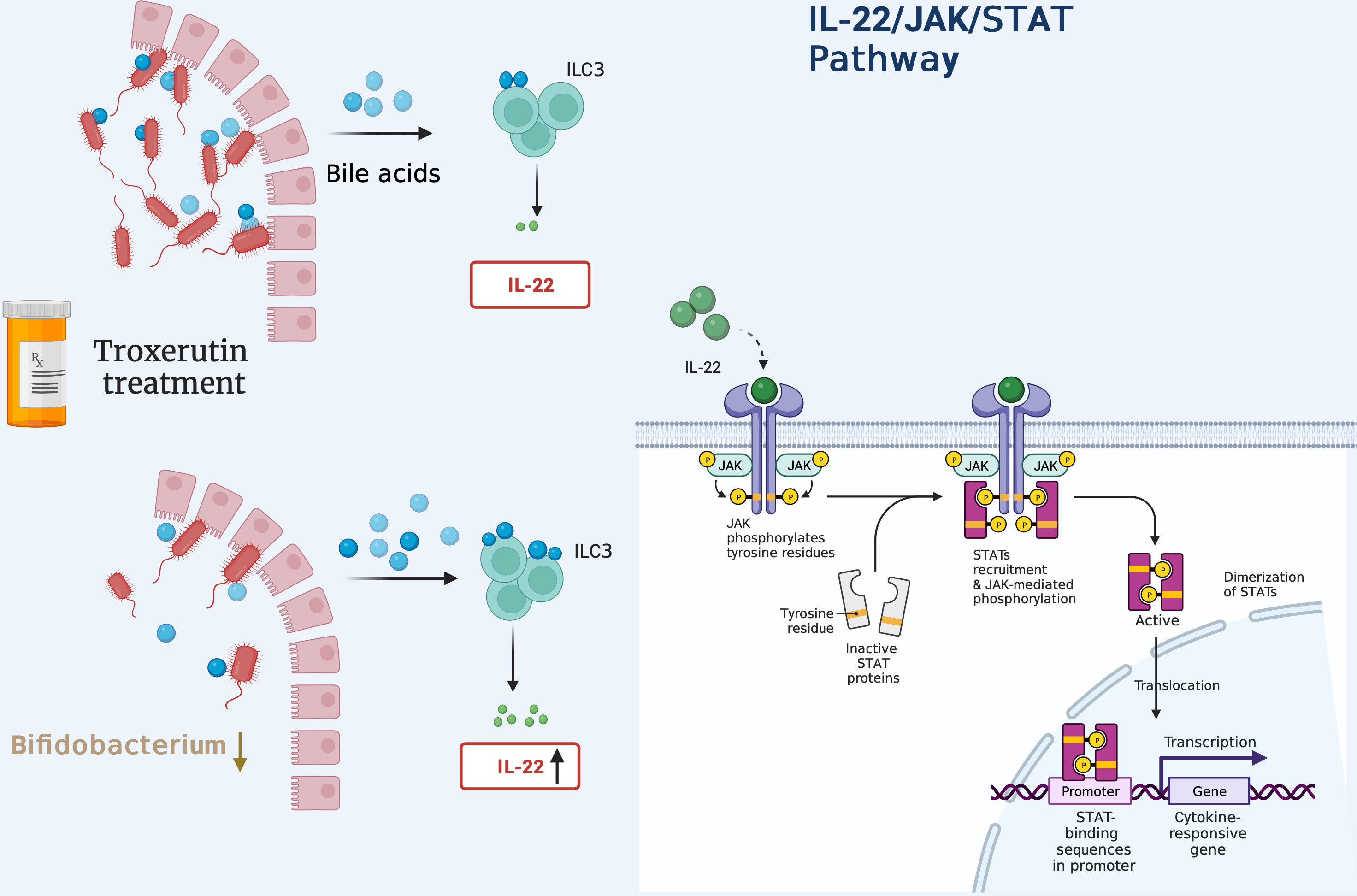
Figure 5 Administration of troxerutin decreases the abundance of Bifidobacterium, inducing the production of more unconjugated secondary bile acids. These bind to TGR5 receptors present in ILC3 cells to secrete more IL-22 in a GATA-dependent manner, thus activating downstream JAK1/STAT3 signaling.
6.7 The Hippo pathway
The Hippo pathway, which was originally discovered in Drosophila, is a highly conserved signaling cascade that plays significant biological roles in controlling organ size, tissue homeostasis, and regeneration (212). The Hippo pathway, unlike other ligand-induced signaling pathways, lacks ligands or receptors (213). The mammalian Hippo pathway cascade is made up of core kinases—mammalian Ste20-like protein kinase ½ (MST1/2) and large tumor suppressor ½ (LATS1/2)—as well as adaptor proteins [Salvador (SAV1) and Mps one binder 1A/B (MOB1A/B)] and the downstream transcriptional co-activators yes-associated protein (YAP) and transcriptional co-activator with PDZ-binding motif (TAZ). The Hippo pathway is modulated by a number of upstream signals, such as mechanical force, G protein-coupled receptors (GPCRs), cell polarity, and cell–cell contact. When the Hippo pathway is active, the MST1/2–SAV1 complex phosphorylates and activates the LATS1/2–MOB1A/B complex, which then directly phosphorylates YAP and TAZ. YAP/TAZ is sequestered in the cytoplasm through 14-3-3 protein binding and subsequently degraded by the proteasome (214). Conversely, when the Hippo pathway is inactive, YAP and TAZ are dephosphorylated and translocate to the nucleus, where they bind with transcription factors (TEADs) to increase the expression of downstream targets: growth factors (CCN2,3) and apoptosis inhibitors (BIRC1,7) (215). These proteins, in turn, stimulate ovarian cell growth and proliferation (216) (Figure 6). Emerging studies have demonstrated the involvement of the Hippo signaling pathway in the regulation of all stages of follicle development (217). The rigid environment of the ovarian cortex activates the Hippo pathway, inhibiting the follicles from entry into the growth phase, thus maintaining the primordial follicles in a dormant state. As follicles grow, they move to the medullar region, which can offer a softer matrix for proliferation and expansion (218). Of note, the modulation of local Hippo signaling in the follicles varies: for example, larger follicles may disrupt the growth of neighboring smaller follicles by enhancing Hippo signaling, resulting in the maturation of a single oocyte. In mice, as the ovary ages, the levels of MST1 and LATS2 declines, while a similar linear correlation is not observed in the case of YAP levels. In addition, premature ovarian failure during later life has observed in LATS1/2-deficient mice.
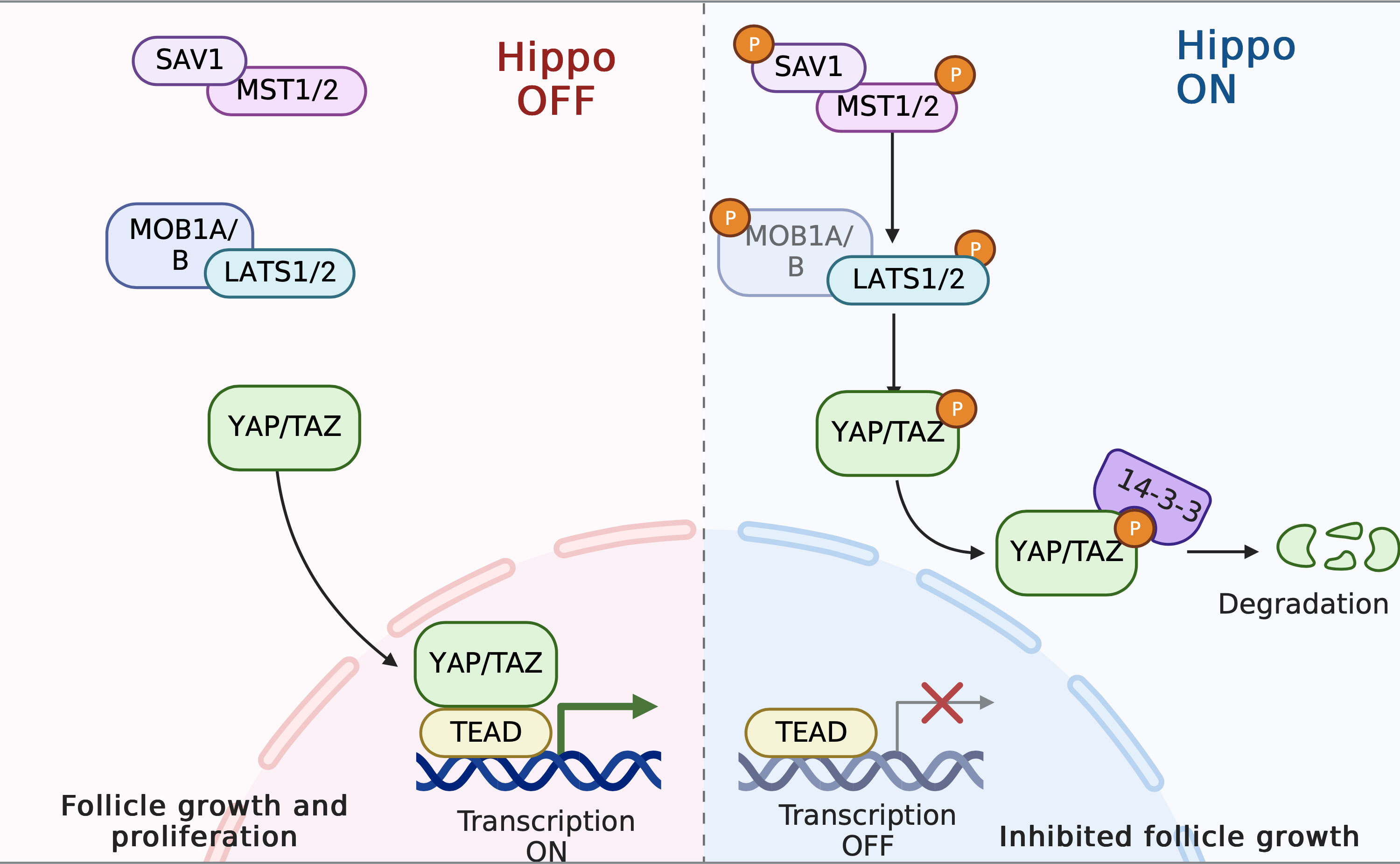
Figure 6 The Hippo pathway in PCOS pathogenesis. When the Hippo pathway is active, the MST1/2–SAV1 complex phosphorylates and activates the LATS1/2–MOB1A/B complex; this then directly phosphorylates YAP and TAZ. Consequently, YAP/TAZ is sequestrated in the cytoplasm by 14-3-3 proteins and subsequently degraded. Follicle growth is inhibited. In contrast, disruption of Hippo signaling leads to nuclear translocation of YAP and increased downstream CCN growth factor transcription, thereby stimulating follicle growth.
It has been demonstrated that disruption of the Hippo signaling pathway is associated with PCOS, premature ovarian failure (POI), and ovarian tumor (219, 220). GWAS have revealed that SNP rs11225161 and rs11225138 from the YAP1 gene are associated with PCOS, specifically with impaired glucose tolerance and high LH levels, respectively (221). Another two loci, rs113168128 (ERBB4) and rs144248326 (WWTR1), have recently been identified and linked to anovulation (222). Of note, similar associations between YAP1 and the SNPs mentioned above have not been detected in teenagers with PCOS (223). Furthermore, one study has found that the YAP1 promoter is hypo-methylated in the GCs of PCOS patients, thus resulting in increased YAP1 mRNA and protein levels. Additionally, this study revealed a negative correlation between the degree of methylation of the YAP1 promoter and androgen levels, in a dose-dependent manner (224). Mechanically, given the sensitivity of Hippo signaling to physical force, the effect of the fibrotic extracellular matrix (ECM) and the thickened and sclerosed cortex of the polycystic ovaries, which contains more F-actin and collagen, is to downregulate Hippo signaling, resulting in YAP1 over-activation, and thus leading to stromal hypertrophy and over-proliferation of theca cells. Finally, this stimulates hyperplastic theca cells to over-produce androgen and causes multiple small immature follicles to be arrested simultaneously (213). Notably, inhibitory YAP1 activity mediated by PKA is required for an LH surge to trigger ovulation (225, 226). In reaction to ovulatory signals, amphiregulin (Areg) initiates transcription under LH pulse stimulation, which triggers the subsequent resumption of meiosis and ovulation. However, Areg concentrations are lower in PCOS, with unchanged LH receptor levels. In addition, it has been revealed that Areg promoter is a direct target of YAP1 (227, 228). Taken together, these findings indicate that the activation of YAP1 is essential for GC proliferation prior to ovulation, but its persistent over-activation may cause anovulation and infertility through interference with AREG expression.
6.7.1 Interplay with AMPK
Compelling evidence has revealed that YAP1/TAZ activity can be modulated by metabolism (229). AMPK is a key energy sensor; it negatively regulates YAP1 activity by activating LATS1/2 through AMOTL1 or by directly phosphorylating YAP1 at Ser 94. Subsequently, YAP1 is sequestered in the cytoplasm and inhibits downstream gene transcription. In concert with prior studies, metformin, a well-known AMPK activator, has been reported to target YAP1 at S127 (230). Findings also indicate that organ or tissue size is precisely controlled and coordinated with energy status. When there is limited energy availability, the Hippo pathway is activated to support basic survival rather than cellular proliferation (231). Under conditions of sufficient nutrients, however, the high level of YAP activity enhances glycolysis and activates mTOR, leading to excessive activation of dormant primordial follicles (154).
6.7.2 Therapeutic strategies
Wedge resection and laparoscopic ovarian drilling (LOD) have been successfully used to treat PCOS by normalizing ovulation (229). It has been found that LOD may loosen cortical layers via alteration of ovarian mechanical forces and destruction of hyperplastic stromal tissue through thermal effects (232), thus decreasing androgen synthesis and normalizing the HPO axis. In vitro activation (IVA) with the administration of actin polymerization—μM jasplakinolide (JASP) or sphingosine-1-phosphate (S1P)—could be a novel therapy for PCOS patients with fewer operative complications. This can convert G-actin into F-actin, increasing YAP nuclear translocation and thereby causing the disrupted follicular growth in PCOS patients to be resumed.
6.8 The Wnt pathway
The Wnt signaling pathway, which is evolutionarily conserved, is also essential for cell proliferation, differentiation, apoptosis, and tissue homeostasis. Dysregulation of this pathway is associated with many types of developmental disorders, fibrosis, and oncogenesis (233). In mammals, there are 19 Wnt ligands, 10 Frizzled (FZD) receptors, and 3 Dishevelled (DVL) proteins. Wnt signaling can be subdivided into two types: β-catenin-dependent signaling (the canonical pathway) and β-catenin-independent signaling (the non-canonical pathway), with the latter further divided into the Wnt/planar cell polarity (PCP) and Wnt/calcium(Ca2+) pathways (234).
6.8.1 Wnt/β-catenin signaling
In the case of Wnt/β-catenin signaling (the canonical pathway), when the ligands (such as Wnt1, Wnt2, Wnt3, Wnt8a, and Wnt10b) bind to the Frizzled (FZD) receptors and LRP5/6 co-receptors, signaling is activated, leading to the recruitment of axis inhibition protein (AXIN1/2) and Dishevelled (DVL), which can inhibit the β-catenin destruction complex (consisting of AXIN, GSK3β, CK1, and APC) (233). The result is that unphosphorylated β-catenin is accumulated and then translocates to the nucleus, where it interacts with T cell-specific factor (TCF)/the lymphoid enhancer factor (LEF) family and recruits co-activators, including CBP/p300 and Bcl-9, thereby activating the expression of WNT target genes such as c-Myc, cyclin D1, and CDKN1A. In the absence of WNT ligands, the signaling pathway is OFF, and the destruction complex phosphorylates β-catenin, leading to ubiquitin–proteasomal degradation (235). Moreover, some antagonists, such as dickkopf-1 (DKK1), secreted Frizzled-related proteins (SFRPs), and WNT modulator in surface ectoderm (WISE), are extracellular modulators of Wnt signaling, inhibiting signaling at the ligand or receptor levels (236).
The roles of the canonical Wnt signaling pathway during follicle formation and maintenance have been widely studied (237). One study has verified the involvement of Wnt/β-catenin signaling in granulosa cell apoptosis in North Chinese women with PCOS. In this investigation, transcriptional levels of WNT1, WNT3, and WNT4 were found to be higher in the PCOS group, whereas levels of SFRP4 were lower. Moreover, survivin and BMP4 (downstream survival-related factors) and β-catenin were dramatically decreased. The level of Bax, an apoptosis-related protein, was significantly higher in the PCOS group than in the control group (238). However, these findings were inconsistent with research by Bicer. In this study, serum SFRP4 levels were found to be elevated in women with PCOS; more specifically, the higher a woman’s level of SFRP4, the greater her risk of developing PCOS (239). As an inflammatory adipocytokine, SFRP4 is associated with the onset of diabetes, both type 1 and type 2. It can decrease the influx of Ca2+ into β pancreatic cells, thereby suppressing the movement of insulin vesicles toward the membrane and impeding insulin release (240). The dynamic changes occurring during culture in vitro and differentiation of GCs could be a reasonable explanation (241). In rat models of PCOS, miR-324-3p inhibits the proliferation of and promotes apoptosis of ovarian granulosa cells by directly targeting WNT2B (242). Similarly, Sanchez and colleagues have also suggested the involvement of the WNT/β-catenin signaling pathway in the luteinized granulosa cell atresia observed in women with endometriosis (243). Another study has investigated the expression profiles of Wnt/β-catenin signaling genes in human oocytes, finding that the expression of WNT1 and GSK3β genes was elevated in PCOS patients, while there was no significant difference between the PCOS group and the control group in terms of APC and β-catenin expression levels. Interestingly, this study did not detect the expression of AXIN2, FZD4, TCF4, WNT5A, WNT3, WNT4, or WNT7A genes in the ovaries of either PCOS patients or healthy women (57). One study has identified the role of FZD3e in FSH-mediated estrogen production (244). Finally, significantly increased FZD3 expression in the cumulus cells of PCOS patients has been found to lead to over-activation of signaling and subsequently more production of stable β-catenin, thus disrupting the recruitment of CYP19A1 promoter (245).
6.8.2 β-catenin-independent signaling
Similar to Wnt/β-catenin signaling, the β-catenin-independent pathway is activated by WNT ligands (such as WNT11 and WNT5A) binding to FZD receptors and ROR1/2 or RYK co-receptors, resulting in activation of downstream effectors (246). One study has observed proinflammatory effects of wnt5a in the GCs of PCOS patients’ ovaries via an interaction between the noncanonical-Ror2 and PI3K/AKT/NF-κB signaling pathways (247). SFRP5 can combine with WNT5a to inhibit the inflammatory process (248). Moreover, inhibition of the NF-κB pathway may disrupt the activation of WNT5A signaling components ROR2 and JNK (249). Similar effects have been thoroughly demonstrated in many studies. For example, WNT-5A/B ligand expression has been found to be increased in the lung fibroblasts of COPD patients, inducing the production of pro-inflammatory cytokines IL-6 and CXCL8 through noncanonical FZD2 signaling (250). The WNT5A ligand is not limited to the noncanonical signaling pathway. Studies have indicated that a switch from canonical to noncanonical WNT signaling can occur. WNT5A can inhibit canonical Wnt signaling activity by competing with the receptor complex, thereby suppressing the secretion of steroid hormones (251, 252).
6.8.3 Crosstalk with Klotho
The Klotho gene is widely recognized to be involved in the anti-aging process in mammals, and under-expression of Klotho is linked to many diseases, including hypertension, diabetes, and chronic kidney disease. The Klotho family includes α-, β-, and γ-Klotho isoforms, which are highly expressed in the kidney but are also found in other tissues such as the liver and AT. Membrane Klotho serves as a co-receptor for many fibroblast growth factors (FGFs) (253). Although exogenous supplementation of Klotho represents a promising treatment for diverse disorders, there is also growing evidence indicating that the over-expression of Klotho and PCOS are closely interrelated. One study measured circulating FGF19, FGF21, and β-Klotho levels in both in PCOS patients and healthy women, finding that β-Klotho levels were significantly higher in PCOS patients; this can be regarded as a strong indicator of PCOS diagnosis (254). In Klotho knock-out mice, the expression level of Wnt1 is increased and the initially inhibited IRS/Akt pathway is restored (255). As mentioned above, these two signaling pathways are both negatively associated with GCs apoptosis. Furthermore, the increased Klotho expression observed in PCOS patients is accompanied by hyperandrogenism. Mechanistically, androgen recruits AR through indirect binding to Klotho promoters at the transcriptional level (256). Meanwhile, other studies have verified that Klotho is expressed in the hypothalamus and the pituitary gland, regulating GH secretion. Of interest, Klotho expression is relatively low in premature ovarian failure (POF), and this upregulates the TGF-β/Smads signaling pathway, which inhibits autophagy. As a consequence, excess ROS disrupts the maturation of oocytes. Hence, it is speculated that the complicated roles played by Klotho are dependent on the signaling pathways in which it participates (257).
6.8.4 Therapeutic strategies
Administration of metformin reverses the low levels of SFRP-5 observed in PCOS patients, and this is expected to be a sensitive indicator for PCOS diagnosis (258). It has reported that CangFu Daotan Decoction normalizes aberrant metabolic features in a rat model of PCOS by suppressing the expression of WNT1 and β-Catenin via modification of m6A methylation (259). Many small molecules or antibody medications have also been found to function as Wnt signaling cascade inhibitors, such as vantictumab (OMP-18R5) and BMD4503-2, which are inhibitors of the Wnt receptor complex; however, development of these focuses primarily on tumor therapy (234, 260). It is believed that, in the near future, more therapeutic strategies targeting Wnt pathway signaling will be developed for PCOS.
6.9 The Notch signaling pathway
The Notch pathway is a highly conserved signaling system. In mammals, it has five ligands (JAG1, JAG2, DLL1, DLL3, and DLL4) and four receptors (NOTCH1, NOTCH2, NOTCH3, and NOTCH4). It exerts physiological effects on the phenotype and functional differentiation of vascular endothelial cells. Recent work has demonstrated that endoplasmic reticulum (ER) stress activates Notch signaling in PCOS, particularly Notch2; ultimately, expression of the downstream proteins Hey2 and Hes1 is increased (261).
6.9.1 Angiogenesis
Angiogenesis in the ovary is critical for follicular growth, ovulation, and regression of the corpus luteum (262). Several abnormalities in ovarian angiogenesis have been identified in women with PCOS, including increased ovarian vascularization and blood flow. The dysregulation of some angiogenic factors, such as vascular endothelial growth factor (VEGF), platelet-derived growth factor (PDGF), transforming growth factor-β (TGFβ), and basic fibroblast growth factor (bFGF), may be partially responsible for ovulation and aberrant cysts in PCOS (263). The VEGF family was the first and is now the most widely studied set of angiogenic factors in the ovaries. Studies have shown that VEGF is positively correlated with ovarian blood flow, and there is an increase in the density of blood vessels in the cortical stroma of the ovaries in PCOS. In contrast, notch ligand DLL4, mainly expressed on the endothelial cells, is a negative regulator of VEGF-mediated microvascular growth and branching via the prevention of excessive branching (264). Using an anti-Dll4 monoclonal antibody in vivo, Fraser and colleagues showed that this resulted in increased luteal angiogenesis and microvascular density; however, these corpora lutea were less functional and regressed earlier (265). Integrated in silico analysis has identified the Notch pathway as being associated with angiogenesis in PCOS, while the PI3K/Akt signaling pathway is the most enriched pathway (266).
6.9.2 MicroRNAs
Previous studies have indicated that certain miRNAs, such as let-7a, miR-221/miR-222, and miR-92b, are involved in follicular development and follicular atresia. Xu et al. identified miR-483-5p as the regulator of Notch3 expression in human cumulus GC (267). Moreover, LINC00173 has been reported to upregulate JAG1 expression and to be involved in the development of PCOS via downregulation of miR-124-3p (268). Considering the easy access to some miRNAs from blood or fluid samples, it is possible that miRNAs could be used as effective tools for PCOS diagnosis at a very early stage (269).
6.9.3 Steroidogenesis
Research has confirmed that the Notch pathway is also essential for steroid hormone synthesis. Studies have found that, when Notch signaling is inhibited in small preantral follicles, the expression of genes in the steroid biosynthetic pathway is upregulated (270). This may have the ability to regulate GATA4-dependent promoters through the notch downstream effectors HEY1, HEY2, and HEYL. GATA4 is a crucial transcription factor for steroidogenesis (271, 272).
6.9.4 Therapeutic strategies
Notch proteins and ligands are abundant in the hippocampus. It has been found that Notch regulates the functions of learning and memory formation. Disruption of Notch signaling has been implicated as a cause of several human diseases, for example, Alzheimer’s disease (AD) (273). The ligand DLL1 has been shown to be upregulated in the brains of AD patients. Liraglutide (LIR) treatment ameliorates cognitive memory impairment in rats with PCOS via downregulation of Notch overexpression, with the mechanism taking the form of increased acetylcholine levels and decreased Aβ aggregation. Additionally, LIR exerts an anti-inflammatory effect through repression of the activation of the NF-κB pathway (274).
6.10 The TGF-β/Smads signaling pathway
The transforming growth factor β (TGF-β) family is crucial for tissue renewal and homeostasis. Its members include molecules such as growth and differentiation factors (GDFs), bone morphogenetic proteins (BMPs), activins, TGF-βs (TGF-β1,2,3), and anti-Müllerian hormone (AMH) (275); their roles in the regulation of ovarian functions have been verified (276). The ligands of the TGF-β family exert their effects by binding to type I or type II receptors (277). In the ligand–receptor complex, type I receptors are phosphorylated by type II receptors, and in turn phosphorylate the R-Smads (Smad2 and Smad3). Subsequently, the activated R-Smads dissociate from the type I receptors and form a complex with co-Smad (Smad4). The complex then translocates to the nucleus, where it regulates the transcription of target genes. In contrast, I-Smads (Smad6 and Smad7), which inhibit the interactions between type I receptors and R-Smads, function as negative regulators of Smad-mediated signaling (278). Studies have indicated that SMAD3, SMAD4, and SMAD5 have close relationships with apoptosis of granulosa cells, the main mechanism underlying follicular atresia (279, 280). TGF-β1 also inhibits the activity of P450 aromatase, an enzyme that converts androgen to estrogen (240, 281).
6.10.1 Fibrosis of the ovary
TGF‐β has three isoforms (TGF‐β1, β2, and β3), with TGF‐β1 being the most common. Many studies have indicated that disruption of the TGF-β1/Smads signaling pathway plays an important role in tissue fibrosis, including renal fibrosis, cardiac fibrosis, and hepatic fibrosis. The mechanism may be due to the transformation of fibroblasts into myofibroblasts, the excessive synthesis of extracellular matrix (ECM), and the inhibition of ECM degradation caused by activation of the TGF-β1/Smads signaling pathway. Moreover, many downstream proteins of TGF-β, such as tissue inhibitor of metalloproteinases (TIMPs), matrix metalloproteinases (MMPs), α-smooth muscle actin (α-SMA), and connective tissue growth factor (CTGF), contribute to organ fibrosis (276). To date, however, few studies have focused on ovarian fibrosis. Recent studies have linked this condition to many ovarian diseases, including PCOS and POI (282). The common features of ovarian fibrosis in PCOS patients are a thickened tunica albuginea, due to increased collagen deposition, and increased cortical stroma. Therefore, the aberrant theca cells produce more steroid hormones (283). TGF-β1 also inhibits the activity of P450 aromatase, an enzyme that converts androgen to estrogen (240, 281). TGF-β1 gene polymorphisms are associated with the development of PCOS and characteristics of women with PCOS in the Korean and Han Chinese population. A study has confirmed that ER stress, a potential determinant of pro-fibrotic remodeling during tissue fibrosis, is activated in the granulosa cells of the ovary in PCOS via induction of TGF-β1 expression (284). Over-activation of the NLRP3 inflammasome, induced by excess androgen, results in significantly increased levels of fibrotic factors, such as TGF-β, CTGF, α-SMA, β-catenin, and the environment becomes more collagenous, with increased expression of collagen I and collagen IV (107). However, it remains unclear whether ovarian fibrosis is the cause of PCOS or the result.
6.10.2 Fibrillin and follistatin
The Fibrillin 3 gene (allele 8 of D19S884) appears to be a susceptibility gene for PCOS and is associated with impaired glucose homeostasis (276). Fibrillin 3 regulates the bioactivity of TGF-β by binding to the TGF-β superfamily ligands. Hence, some variants of the Fibrillin gene are thought to alter the normal function of TGF-β signaling and contribute to the pathogenesis of PCOS (285). Elevated follistatin levels have been detected in PCOS patients, regardless of weight. As this is an activin-binding protein, increased follistatin levels result in the neutralization of more activin, which is capable of stimulating FSH secretion. Accordingly, this leads to more androgen production by theca cells and disrupts follicular development (286, 287).
6.10.3 Growth differentiation factors
As mentioned above, GDF8 also belongs to the TGF-β superfamily and plays crucial roles in the regulation of folliculogenesis, in steroidogenesis, and in luteal function (288). As an intra-ovarian factor, a number of studies have linked dysregulated GDF8 to many types of reproductive disorders, such as ovarian hyperstimulation syndrome (OHSS) and PCOS. GDF-8 expression levels in follicular fluid and in serum tend to be elevated in PCOS patients, although it is noteworthy that this effect is only observed in obese PCOS patients (289). Moreover, elevated GDF8 levels in women with PCOS often function as a predictor of poorer pregnancy outcomes when women are undergoing IVF treatment (290). One study has revealed that GDF8 is the culprit for the abnormal glucose metabolism observed in PCOS as a result of its stimulation of the expression of SERPINE1 through the ALK5-mediated SMAD2/3-SMAD4 signaling pathway. The SERPINE1 gene encodes plasminogen activator inhibitor 1 (PAI-1), leading to hypofibrinolysis, and is closely associated with diabetes and IR (291, 292). Intriguingly, unlike GDF-8, there is no obvious difference between normal and polycystic ovaries in the expression of GDF11, which has a similar molecular structure and function to GDF8 (293).
6.10.4 Therapeutic strategies
6.10.4.1 Small molecules
One study has proven that a potent ALK5 inhibitor, namely SB431542, can inhibit related molecules, such as TGF-β, Smad3, Smad2, and a-SMA, and upregulates anti-fibrotic factor MMP2 in rats with DHEA-induced PCOS, thereby mitigating ovarian fibrosis via the TGF-β/Smads signaling pathway (283). Moreover, its value in improving ovarian morphology in PCOS has been verified (291). Given the high levels of GDF8 and GDF15 observed in patients with PCOS, weight management measures, such as exercises, training, and a ketogenic diet, appear to be a good option (294–296).
6.10.5 Drugs
6.10.5.1 Rosiglitazone
Rosiglitazone, a PPAR-γ agonist, may alleviate ovarian fibrosis by suppressing the transduction of TGF-β1 and lowering CTGF levels in a rat model of PCOS (282). PPAR-γ has been found to inhibit nuclear translocation of the transcription factor NF-κB and activator protein-1 (AP-1), thus serving anti-inflammatory and anti-fibrotic roles (297).
6.10.5.2 Sitagliptin
The dipeptidyl peptidase-4 (DPP4) inhibitor sitagliptin is widely used to treat type 2 diabetes, but researchers have recently found that it also has a therapeutic effect in renal and hepatic fibrosis. A study has demonstrated that sitagliptin can suppress ovarian fibrosis in rats with PCOS through downregulation of the TGF‐β1/Smad2/3 signaling pathway (298).
6.10.6 Phytochemicals
6.10.6.1 Proanthocyanidins
PCs, derived from many dark-green leafy vegetables, improve ovarian fibrosis in mice with PCOS through regulation of serum hormone levels, inhibiting oxidative stress and suppressing activation of the TGF-β1/Smads signaling pathway (299).
6.10.6.2 Paeoniflorin
PAE, a major active component of Paeonia lactiflora Pallas, has potent anti-inflammatory and immune-regulatory effects (300). Zhou et al. have shown that PAE may reduce the expression of TGF-β1 and Smad3, while increasing the expression of Smad7 and MMP2 (a negative regulator of the TGF-β1/Smads signaling pathway), especially at a high dose of PAE, in rat models (301). These findings are consistent with previous studies on the effects of PAE in the treatment of liver fibrosis, myocardial fibrosis, and lung fibrosis.
6.10.6.3 Rhamnocitrin
Rha has also been discovered to downregulate the TGF-β1/Smads signaling pathway and to suppress NF-κB through activation of PPAR-γ (302, 303).
6.10.6.4 Resveratrol
Regulation of the TGF-β signaling pathway and ROS are interconnected. ROS can stimulate the TGFβ ligand and promote the expression of fibroblast TGF-β; in turn, TGF-β induces an increase in ROS in certain tumor cells (304). Resveratrol exerts anti-fibrotic and anti-apoptotic effects through inhibition of p66Shc-mediated ROS production and expression of fibrotic factors (305, 306).
6.11 The Hedgehog signaling pathway
Hedgehog (Hh) signaling has been implicated in a regulatory role in the developmental processes of both embryo and adult tissues. Abnormal activation of Hh signaling has been linked to numerous types of cancer. The mammalian hedgehog family consists of three ligands [sonic hedgehog (SHH), desert hedgehog (DHH), and Indian hedgehog (IHH)], two receptors (PTCH1 and PTCH2), and the seven-transmembrane signal transducer protein Smoothened (SMO). If there is no ligand, PTCH1 or PTCH2 inhibits the activity of SMO. Once the ligand binds to the receptor, SMO inhibition is lifted, leading to the activation of downstream transcription factors, namely glioma-associated oncogene homologs (Gli1, Gli2, and Gli3), in the nucleus (307). Many studies have demonstrated its impact on follicle growth and the proliferation of theca and granulosa cells (308). A lack of Dhh/Ihh in the ovaries causes theca cell loss, disrupted steroid production, and failure to form the corpora lutea (309). According to pathway analysis based on genome-wide methylation profiling in the granulosa lutein cells of PCOS patients, the Hedgehog pathway is associated with the development of PCOS (310).
6.11.1 Steroidogenesis
One of the main characteristics of PCOS patients is HA, and the ovarian theca cells are thought to be one of the major sources of excess androgen in PCOS patients (311). Liu et al. have demonstrated that establishment of a theca cell lineage requires both granulosa cells and oocytes, with involvement through multicellular interactions via GDF9 and Hh signaling (309). Interestingly, HH ligands (DHH and IHH) are expressed in the granulosa cells of primary-to-antral-stage follicles, whereas the downstream effector Gli1 is found in the theca layer (312). Moreover, expression of Srd5a3 and Cyp17a1, which are steroidogenesis-related genes, has been found to be decreased in Ihh single-knockout mice. This implies a role for Ihh in the regulation of steroidogenesis in the ovary, and the same study also proved that Ihh has greater influence on the activation of the Hedgehog signaling pathway (313).
6.11.2 Follicular growth and anovulation
In the absence of Dhh and Ihh, the theca cell layer fails to form and preantral follicles are unable to develop. Li observed higher levels of Ihh and Ptch2 in PCOS patients based on RT-PCR analysis when compared to a control group, indicating over-expression of the Hh signaling pathway in PCOS patients. This is also related to the aberrant follicular growth observed in PCOS patients (314). Yi et al. have demonstrated that overactivation of the Hedgehog signaling pathway in the ovary inhibits ovulation. In their experiment, transgenic mice expressing a dominant active allele of the signal transducer Smoothened (SmoM2) were able to prevent the suppression of its activity by PTCH, thus leading to sustaining activation of Hedgehog signaling. The Amhr2cre/+SmoM2 mice exhibited extremely reduced ovulation. This may be attributable to impaired muscle cells or contractile cells in the theca layer, which are very important for ovulation (315, 316). Another study has demonstrated that the anovulatory follicles of mhr2cre/+SmoM2 mutant mice are caused by denser capillaries in the ovarian cortex and a lack of vascular smooth muscle in the theca cells of such mice (317). Therefore, it is very likely that disrupted SH signaling contributes to the development of anovulation in PCOS (311). High levels of GTPase immunity-associated protein (GIMAP) 7 expression is observed in the GCs of PCOS patients. In contrast, silencing of the GIMAP7 gene protects GCs from apoptosis and OS via upregulation of the SHH signaling pathway. The expression of downstream components SHH and SMO is increased, along with increased levels of the antioxidants SOD and GSH (318).
6.11.3 Therapeutic strategies
The Hh signaling pathway inhibitor cyclopamine can decrease apoptosis of ovarian granulosa cells in PCOS (314). Conversely, another study has revealed that administration of cyclopamine partially counteracts the protective effects of GIMAP7 knockout on rats with PCOS with increased ROS levels (318). Further studies focusing on the impact of different Hh ligands on PCOS development are warranted. Moreover, exposure to certain chemicals, such as acetazolamide and itraconazole, as well as maternal smoking, can disrupt HH signaling, thereby interfering with the development of follicles and contributed to infertility to some extent (319). More attention needs to be paid to the avoidance of exposure to these substances.
7 Conclusion and future directions
As it is an intricately complex disorder, multiple signaling pathways and components are involved in the pathophysiological processes of PCOS, and intriguing interactions occur between each signaling cascade. These pathways collectively regulate metabolic and ovarian functions, including folliculogenesis, steroidogenesis, angiogenesis, oocyte maturation, and ovulation. Dysregulation of these pathways contributes jointly to the pathological states involved in PCOS, such as IR, HA, OS, and inflammation (Figure 7). Moreover, some molecules (for instance, SFRP-5, β-Klotho, leptins, and certain miRNAs) are considered to be promising diagnostic biomarkers for PCOS. We have outlined the relevant signal transduction pathways involved in the development of PCOS as well as the crosstalk between them. Inspiringly, several bioactive compounds derived from herbs and TCM have exhibited unique advantages in PCOS treatment in recent years. In Table 1, we list current pharmacological approaches targeting the signaling pathways involved in order to reverse PCOS symptoms and restore ovarian functions in animal models or at the cellular level. However, until now, the options for agents and supplements to treat this condition have been limited, with most such treatments being off-label. Fortunately, the efficacy of some of these interventions has been evaluated in clinical trials, which have shown promising results in the management of PCOS. In Table 2, we present an investigation and summary of glucose-lowering agents, dietary supplements (including minerals, e.g., chromium, zinc, calcium, and magnesium; vitamins; and other nutrients, e.g., herbs and probiotic and polyphenolic compounds), and in Table 3, we list some non-pharmacological treatments for PCOS in view of these clinical trials.
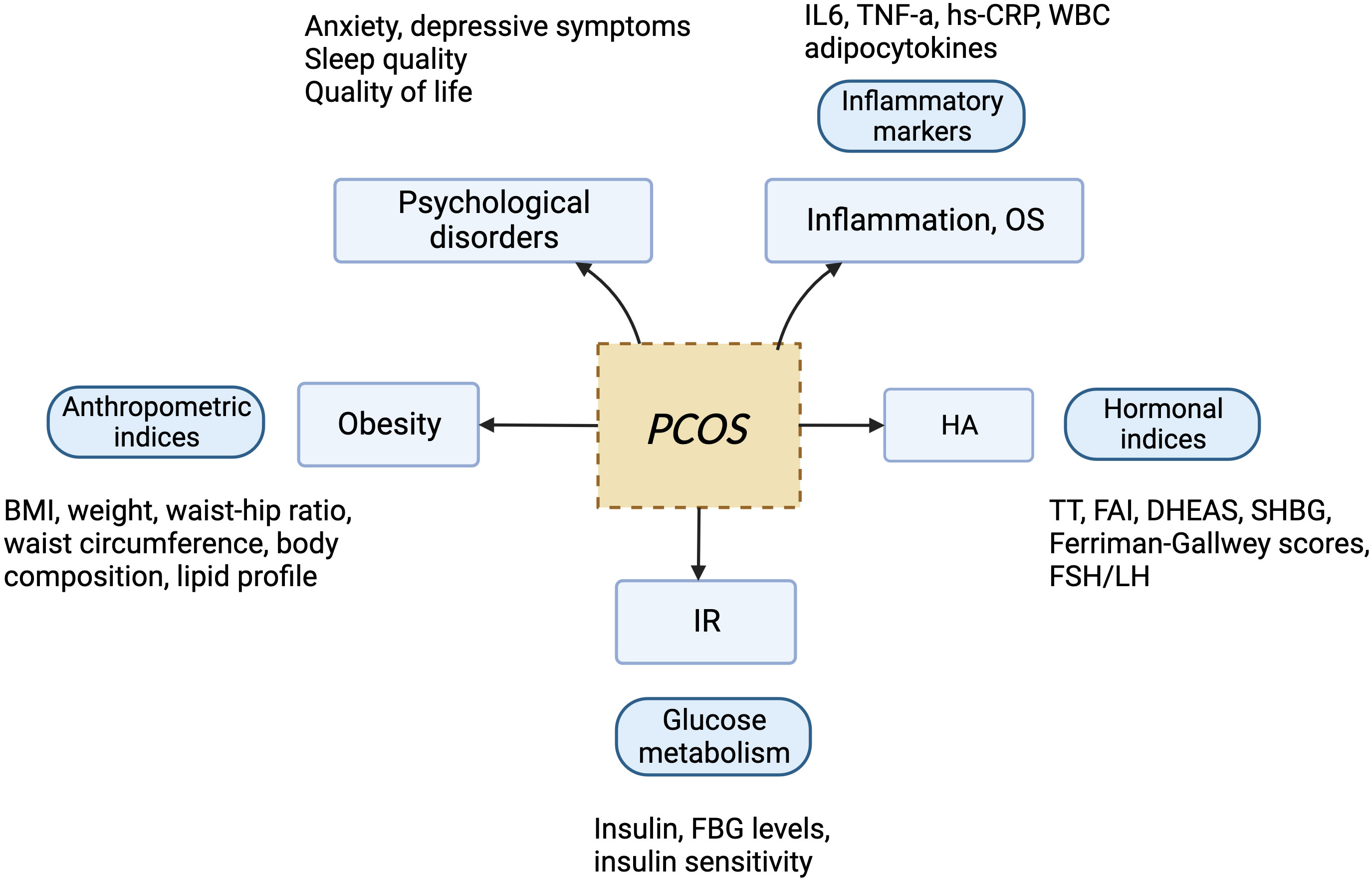
Figure 7 The effects of drugs, dietary supplements, and non-pharmacological treatments on the main health outcomes associated with PCOS. HA, hyperandrogenism; IR, insulin resistance; TT, total testosterone; SHBG, sex hormone-binding globulin; FAI, free androgen index; FBG, fasting blood glucose; BMI, body mass index.

Table 1 Summary of pharmacological therapies targeting signaling pathways involved in PCOS pathogenesis in animal models.
Since little is known about this disease, many women suffer from infertility, hirsutism, irregular menstrual periods, psychological disorders, and long-term complications brought about by PCOS throughout their lives. More in-depth research and greater attention to PCOS are required in order to solve the mystery of this condition.
Author contributions
KW wrote the original manuscript and completed the figures and tables. YL helped write and revise this paper. All authors contributed to the article and approved the submitted version.
Conflict of interest
The authors declare that the research was conducted in the absence of any commercial or financial relationships that could be construed as a potential conflict of interest.
Publisher’s note
All claims expressed in this article are solely those of the authors and do not necessarily represent those of their affiliated organizations, or those of the publisher, the editors and the reviewers. Any product that may be evaluated in this article, or claim that may be made by its manufacturer, is not guaranteed or endorsed by the publisher.
References
1. Teede HJ, Misso ML, Costello MF, Dokras A, Laven J, Moran L, et al. Recommendations from the international evidence-based guideline for the assessment and management of polycystic ovary syndrome. Hum Reprod (2018) 33(9):1602–18. doi: 10.1093/humrep/dey256
2. Chang S, Dunaif A. Diagnosis of polycystic ovary syndrome: which criteria to use and when? Endocrinol Metab Clin North Am (2021) 50(1):11–23. doi: 10.1016/j.ecl.2020.10.002
3. Huddleston HG, Dokras A. Diagnosis and treatment of polycystic ovary syndrome. JAMA (2022) 327(3):274–5. doi: 10.1001/jama.2021.23769
4. Dapas M, Lin FTJ, Nadkarni GN, Sisk R, Legro RS, Urbanek M, et al. Distinct subtypes of polycystic ovary syndrome with novel genetic associations: An unsupervised, phenotypic clustering analysis. PloS Med (2020) 17(6):e1003132. doi: 10.1371/journal.pmed.1003132
5. Szeliga A, Rudnicka E, Maciejewska-Jeske M, Kucharski M, Kostrzak A, Hajbos M, et al. Neuroendocrine determinants of polycystic ovary syndrome. Int J Environ Res Public Health (2022) 19(5):3089. doi: 10.3390/ijerph19053089
6. Walters KA, Gilchrist RB, Ledger WL, Teede HJ, Handelsman DJ, Campbell RE. New perspectives on the pathogenesis of PCOS: neuroendocrine origins. Trends Endocrinol Metab (2018) 29(12):841–52. doi: 10.1016/j.tem.2018.08.005
7. Moore AM. Impaired steroid hormone feedback in polycystic ovary syndrome: Evidence from preclinical models for abnormalities within central circuits controlling fertility. Clin Endocrinol (Oxf) (2022) 97(2):199–207. doi: 10.1111/cen.14711
8. Okada H, Kanasaki H, Tumurbaatar T, Tumurgan Z, Oride A, Kyo S. Hyperandrogenism induces proportional changes in the expression of Kiss-1, Tac2, and DynA in hypothalamic KNDy neurons. Reprod Biol Endocrinol (2022) 20(1):91. doi: 10.1186/s12958-022-00963-w
9. Hu KL, Chen Z, Li X, Cai E, Yang H, Chen Y, et al. Advances in clinical applications of kisspeptin-GnRH pathway in female reproduction. Reprod Biol Endocrinol (2022) 20(1):81. doi: 10.1186/s12958-022-00953-y
10. Moghetti P, Tosi F. Insulin resistance and PCOS: chicken or egg? J Endocrinol Invest (2021) 44(2):233–44. doi: 10.1007/s40618-020-01351-0
11. Herman R, Sikonja J, Jensterle M, Janez A, Dolzan V. Insulin metabolism in polycystic ovary syndrome: secretion, signaling, and clearance. Int J Mol Sci (2023) 24(4):3140. doi: 10.3390/ijms24043140
12. Stepto NK, Cassar S, Joham AE, Hutchison SK, Harrison CL, Goldstein RF, et al. Women with polycystic ovary syndrome have intrinsic insulin resistance on euglycaemic-hyperinsulaemic clamp. Hum Reprod (2013) 28(3):777–84. doi: 10.1093/humrep/des463
13. Hansen SL, Svendsen PF, Jeppesen JF, Hoeg LD, Andersen NR, Kristensen JM, et al. Molecular mechanisms in skeletal muscle underlying insulin resistance in women who are lean with polycystic ovary syndrome. J Clin Endocrinol Metab (2019) 104(5):1841–54. doi: 10.1210/jc.2018-01771
14. Liu Q, Tang B, Zhu Z, Kraft P, Deng Q, Stener-Victorin E, et al. A genome-wide cross-trait analysis identifies shared loci and causal relationships of type 2 diabetes and glycaemic traits with polycystic ovary syndrome. Diabetologia (2022) 65(9):1483–94. doi: 10.1007/s00125-022-05746-x
15. Li X, Xiao H, Ma Y, Zhou Z, Chen D. Identifying novel genetic loci associated with polycystic ovary syndrome based on its shared genetic architecture with type 2 diabetes. Front Genet (2022) 13:905716. doi: 10.3389/fgene.2022.905716
16. Amin M, Gragnoli C. Genome-wide linkage and association study identifies novel genes and pathways implicated in polycystic ovarian syndrome. Eur Rev Med Pharmacol Sci (2023) 27(8):3719–32. doi: 10.26355/eurrev_202304_32171
17. Azziz R, Carmina E, Chen Z, Dunaif A, Laven JSE, Legro RS, et al. Polycystic ovary syndrome. Nat Rev Dis Primers (2016) 2:16057. doi: 10.1038/nrdp.2016.57
18. Tay CT, Garrad R, Mousa A, Bahri M, Joham A, Teede H. Polycystic ovary syndrome (PCOS): international collaboration to translate evidence and guide future research. J Endocrinol (2023) 257(3):e220232. doi: 10.1530/JOE-22-0232
19. Armanini D, Boscaro M, Bordin L, Sabbadin C. Controversies in the pathogenesis, diagnosis and treatment of PCOS: focus on insulin resistance, inflammation, and hyperandrogenism. Int J Mol Sci (2022) 23(8):4110. doi: 10.3390/ijms23084110
20. Ye W, Xie T, Song Y, Zhou L. The role of androgen and its related signals in PCOS. J Cell Mol Med (2021) 25(4):1825–37. doi: 10.1111/jcmm.16205
21. Emanuel RHK, Roberts J, Docherty PD, Lunt H, Campbell RE, Möller K. A review of the hormones involved in the endocrine dysfunctions of polycystic ovary syndrome and their interactions. Front Endocrinol (Lausanne) (2022) 13:1017468. doi: 10.3389/fendo.2022.1017468
22. Auer MK, Hawley JM, Lottspeich C, Bidlingmaier M, Sappl A, Nowotny HF, et al. 11-Oxygenated androgens are not secreted by the human ovary: in-vivo data from four different cases of hyperandrogenism. Eur J Endocrinol (2022) 187(6):K47–53. doi: 10.1530/EJE-22-0518
23. O’Reilly MW, Kempegowda P, Jenkinson C, Taylor AE, Quanson JL, Storbeck K-H, et al. 11-oxygenated C19 steroids are the predominant androgens in polycystic ovary syndrome. J Clin Endocrinol Metab (2017) 102(3):840–8. doi: 10.1210/jc.2016-3285
24. Walzer D, Turcu AF, Jha S, Abel BS, Auchus RJ, Merke DP, et al. Excess 11-oxygenated androgens in women with severe insulin resistance are mediated by adrenal insulin receptor signaling. J Clin Endocrinol Metab (2022) 107(9):2626–35. doi: 10.1210/clinem/dgac365
25. Cirillo F, Catellani C, Lazzeroni P, Sartori C, Tridenti G, Vezzani C, et al. HMGB1 is increased in adolescents with polycystic ovary syndrome (PCOS) and decreases after treatment with myo-inositol (MYO) in combination with alpha-lipoic acid (ALA). Gynecol Endocrinol (2020) 36(7):588–93. doi: 10.1080/09513590.2020.1725967
26. Paulukinas RD, Mesaros CA, Penning TM. Conversion of classical and 11-oxygenated androgens by insulin-induced AKR1C3 in a model of human PCOS adipocytes. Endocrinology (2022) 163(7):bqac068. doi: 10.1210/endocr/bqac068
27. Paulukinas RD, Penning TM. Insulin-induced AKR1C3 induces fatty acid synthase in a model of human PCOS adipocytes. Endocrinology (2023) 164(5):bqad033. doi: 10.1210/endocr/bqad033
28. Kim JJ, Kim D, Yim JY, Kang JH, Han KH, Kim SM, et al. Polycystic ovary syndrome with hyperandrogenism as a risk factor for non-obese non-alcoholic fatty liver disease. Aliment Pharmacol Ther (2017) 45(11):1403–12. doi: 10.1111/apt.14058
29. Persson S, Elenis E, Turkmen S, Kramer MS, Yong EL, Poromaa IS. Higher risk of type 2 diabetes in women with hyperandrogenic polycystic ovary syndrome. Fertil Steril (2021) 116(3):862–71. doi: 10.1016/j.fertnstert.2021.04.018
30. Mukherjee AG, Wanjari UR, Kannampuzha S, Murali R, Namachivayam A, Ganesan R, et al. The implication of mechanistic approaches and the role of the microbiome in polycystic ovary syndrome (PCOS): A review. Metabolites (2023) 13(1):129. doi: 10.3390/metabo13010129
31. Ruth KS, Day FR, Tyrrell J, Thompson DJ, Wood AR, Mahajan A, et al. Using human genetics to understand the disease impacts of testosterone in men and women. Nat Med (2020) 26(2):252–8. doi: 10.1038/s41591-020-0751-5
32. Risal S, Pei Y, Lu H, Manti M, Fornes R, Pui H-P, et al. Prenatal androgen exposure and transgenerational susceptibility to polycystic ovary syndrome. Nat Med (2019) 25(12):1894–904. doi: 10.1038/s41591-019-0666-1
33. Zhang HL, Yi M, Li D, Li R, Zhao Y, Qiao J. Transgenerational inheritance of reproductive and metabolic phenotypes in PCOS rats. Front Endocrinol (Lausanne) (2020) 11:144. doi: 10.3389/fendo.2020.00144
34. Stener-Victorin E. Update on animal models of polycystic ovary syndrome. Endocrinology (2022) 163(12):bqac164. doi: 10.1210/endocr/bqac164
35. Stener-Victorin E, Padmanabhan V, Walters KA, Campbell RE, Benrick A, Giacobini P, et al. Animal models to understand the etiology and pathophysiology of polycystic ovary syndrome. Endocr Rev (2020) 41(4):bnaa010. doi: 10.1210/endrev/bnaa010
36. Dabravolski SA, Nikiforov NG, Eid AH, Nedosugova LV, Starodubova AV, Popkova TV, et al. Mitochondrial dysfunction and chronic inflammation in polycystic ovary syndrome. Int J Mol Sci (2021) 22(8):3923. doi: 10.3390/ijms22083923
37. Rostamtabar M, Esmaeilzadeh S, Tourani M, Rahmani A, Baee M, Shirafkan F, et al. Pathophysiological roles of chronic low-grade inflammation mediators in polycystic ovary syndrome. J Cell Physiol (2021) 236(2):824–38. doi: 10.1002/jcp.29912
38. He Z, Wang Y, Zhuan L, Li Y, Tang Z-O, Wu Z, et al. MIF-mediated NF-κB signaling pathway regulates the pathogenesis of polycystic ovary syndrome in rats. Cytokine (2021) 146:155632. doi: 10.1016/j.cyto.2021.155632
39. Lu J, Wang Z, Cao J, Chen Y, Dong Y. A novel and compact review on the role of oxidative stress in female reproduction. Reprod Biol Endocrinol (2018) 16(1):80. doi: 10.1186/s12958-018-0391-5
40. Fox CW, Zhang L, Sohni A, Doblado M, Wilkinson MF, Chang RJ, et al. Inflammatory stimuli trigger increased androgen production and shifts in gene expression in theca-interstitial cells. Endocrinology (2019) 160(12):2946–58. doi: 10.1210/en.2019-00588
41. Murri M, Luque-Ramírez M, Insenser M, Ojeda-Ojeda M, Escobar-Morreale HF. Circulating markers of oxidative stress and polycystic ovary syndrome (PCOS): a systematic review and meta-analysis. Hum Reprod Update (2013) 19(3):268–88. doi: 10.1093/humupd/dms059
42. Barrea L, Marzullo P, Muscogiuri G, Di Somma C, Scacchi M, Orio F, et al. Source and amount of carbohydrate in the diet and inflammation in women with polycystic ovary syndrome. Nutr Res Rev (2018) 31(2):291–301. doi: 10.1017/S0954422418000136
43. Li YZ, Di Cristofano A, Woo M. Metabolic role of PTEN in insulin signaling and resistance. Cold Spring Harb Perspect Med (2020) 10(8):a036137. doi: 10.1101/cshperspect.a036137
44. Ferreira AS, Macedo C, Silva AM, Delerue-Matos C, Costa P, Rodrigues F. Natural products for the prevention and treatment of oral mucositis-A review. Int J Mol Sci (2022) 23(8):4385. doi: 10.3390/ijms23084385
45. Anjali G, Kaur S, Lakra R, Taneja J, Kalsey GS, Nagendra A, et al. FSH stimulates IRS-2 expression in human granulosa cells through cAMP/SP1, an inoperative FSH action in PCOS patients. Cell Signal (2015) 27(12):2452–66. doi: 10.1016/j.cellsig.2015.09.011
46. Chahal N, Geethadevi A, Kaur S, Lakra R, Nagendra A, Shrivastav TG, et al. Direct impact of gonadotropins on glucose uptake and storage in preovulatory granulosa cells: Implications in the pathogenesis of polycystic ovary syndrome. Metabolism (2021) 115:154458. doi: 10.1016/j.metabol.2020.154458
47. Ezeh U, Chen IYD, Chen YH, Azziz R. Adipocyte expression of glucose transporter 1 and 4 in PCOS: Relationship to insulin-mediated and non-insulin-mediated whole-body glucose uptake. Clin Endocrinol (Oxf) (2019) 90(4):542–52. doi: 10.1111/cen.13931
48. Jensterle M, Kravos NA, Dolžan V, Goričar K, Herman R, Rizzo M, et al. Glucose transporter 4 mRNA expression in subcutaneous adipose tissue of women with PCOS remains unchanged despite metformin withdrawal: is there a cellular metabolic treatment legacy effect? Endocrine (2022) 75(3):804–13. doi: 10.1007/s12020-021-02934-4
49. Kumariya S, Ubba V, Jha RK, Gayen JR. Autophagy in ovary and polycystic ovary syndrome: role, dispute and future perspective. Autophagy (2021) 17(10):2706–33. doi: 10.1080/15548627.2021.1938914
50. Yang JL, Zhang CP, Li L, Huang L, Ji SY, Lu CL, et al. Testosterone induces redistribution of forkhead box-3a and down-regulation of growth and differentiation factor 9 messenger ribonucleic acid expression at early stage of mouse folliculogenesis. Endocrinology (2010) 151(2):774–82. doi: 10.1210/en.2009-0751
51. Xu R, Wang Z. Involvement of transcription factor foxO1 in the pathogenesis of polycystic ovary syndrome. Front Physiol (2021) 12:649295. doi: 10.3389/fphys.2021.649295
52. Zhang S, Deng W, Liu Q, Wang P, Yang W, Ni W. Altered m6 A modification is involved in up-regulated expression of FOXO3 in luteinized granulosa cells of non-obese polycystic ovary syndrome patients. J Cell Mol Med (2020) 24(20):11874–82. doi: 10.1111/jcmm.15807
53. Miao H, Zhang Y, Lu Z, Liu Q, Gan L. FOXO1 involvement in insulin resistance-related pro-inflammatory cytokine production in hepatocytes. Inflammation Res (2012) 61(4):349–58. doi: 10.1007/s00011-011-0417-3
54. Li N, Wang X, Wang X, Yu H, Lin L, Sun C, et al. Upregulation of foxO 1 signaling mediates the proinflammatory cytokine upregulation in the macrophage from polycystic ovary syndrome patients. Clin Lab (2017) 63(2):301–11. doi: 10.7754/Clin.Lab.2016.160514
55. Wang D, Guo Y, Chai S, Shen K, Li Y, Zhao R. Expression of angiopoietin-like protein 2 in ovarian tissue of rat polycystic ovarian syndrome model and its correlation study. Reprod Biol Endocrinol (2020) 18(1):94. doi: 10.1186/s12958-020-00651-7
56. Chang W, Goodarzi MO, Williams H, Magoffin DA, Pall M, Azziz R. Adipocytes from women with polycystic ovary syndrome demonstrate altered phosphorylation and activity of glycogen synthase kinase 3. Fertil Steril (2008) 90(6):2291–7. doi: 10.1016/j.fertnstert.2007.10.025
57. Ismail AB, Naji M ‘Mohammad S, Nebih İ, Tuncel G, Ozbakir B, Temel SG, et al. The expression profile of WNT/β-catanin signalling genes in human oocytes obtained from polycystic ovarian syndrome (PCOS) patients. Zygote (2022) 30(4):536–42. doi: 10.1017/S0967199422000028
58. Zhang Y, Sun X, Sun X, Meng F, Hu M, Li X, et al. Molecular characterization of insulin resistance and glycolytic metabolism in the rat uterus. Sci Rep (2016) 6:30679. doi: 10.1038/srep30679
59. Shafiee MN, Seedhouse C, Mongan N, Chapman C, Deen S, Abu J, et al. Up-regulation of genes involved in the insulin signalling pathway (IGF1, PTEN and IGFBP1) in the endometrium may link polycystic ovarian syndrome and endometrial cancer. Mol Cell Endocrinol (2016) 424:94–101. doi: 10.1016/j.mce.2016.01.019
60. Gao Y, Chen J, Ji R, Ding J, Zhang Y, Yang J. USP25 regulates the proliferation and apoptosis of ovarian granulosa cells in polycystic ovary syndrome by modulating the PI3K/AKT pathway via deubiquitinating PTEN. Front Cell Dev Biol (2021) 9:779718. doi: 10.3389/fcell.2021.779718
61. Zhu Q, Yao Y, Xu L, Wu H, Wang W, He Y, et al. Elevated SAA1 promotes the development of insulin resistance in ovarian granulosa cells in polycystic ovary syndrome. Reprod Biol Endocrinol (2022) 20(1):4. doi: 10.1186/s12958-021-00873-3
62. Zhong X, Ke C, Cai Z, Wu H, Ye Y, Liang X, et al. LNK deficiency decreases obesity-induced insulin resistance by regulating GLUT4 through the PI3K-Akt-AS160 pathway in adipose tissue. Aging (Albany NY) (2020) 12(17):17150–66. doi: 10.18632/aging.103658
63. Hao M, Yuan F, Jin C, Zhou Z, Cao Q, Xu L, et al. Overexpression of lnk in the ovaries is involved in insulin resistance in women with polycystic ovary syndrome. Endocrinology (2016) 157(10):3709–18. doi: 10.1210/en.2016-1234
64. Tan M, Cheng Y, Zhong X, Yang D, Jiang S, Ye Y, et al. LNK promotes granulosa cell apoptosis in PCOS via negatively regulating insulin-stimulated AKT-FOXO3 pathway. Aging (Albany NY) (2021) 13(3):4617–33. doi: 10.18632/aging.202421
65. Saliminejad K, Khorram Khorshid HR, Soleymani Fard S, Ghaffari SH. An overview of microRNAs: Biology, functions, therapeutics, and analysis methods. J Cell Physiol (2019) 234(5):5451–65. doi: 10.1002/jcp.27486
66. Zhou Z, Tu Z, Zhang J, Tan C, Shen X, Wan B, et al. Follicular fluid-derived exosomal microRNA-18b-5p regulates PTEN-mediated PI3K/akt/mTOR signaling pathway to inhibit polycystic ovary syndrome development. Mol Neurobiol (2022) 59(4):2520–31. doi: 10.1007/s12035-021-02714-1
67. Chen H, Fu Y, Guo Z, Zhou X. MicroRNA-29c-3p participates in insulin function to modulate polycystic ovary syndrome via targeting Forkhead box O 3. Bioengineered (2022) 13(2):4361–71. doi: 10.1080/21655979.2022.2033014
68. Zhang SW, Zhou J, Gober HJ, Leung WT, Wang L. Effect and mechanism of berberine against polycystic ovary syndrome. BioMed Pharmacother (2021) 138:111468. doi: 10.1016/j.biopha.2021.111468
69. Zhang N, Liu X, Zhuang L, Liu X, Zhao H, Shan Y, et al. Berberine decreases insulin resistance in a PCOS rats by improving GLUT4: Dual regulation of the PI3K/AKT and MAPK pathways. Regul Toxicol Pharmacol (2020) 110:104544. doi: 10.1016/j.yrtph.2019.104544
70. Zhao H, Zhou D, Chen Y, Liu D, Chu S, Zhang S. Beneficial effects of Heqi san on rat model of polycystic ovary syndrome through the PI3K/AKT pathway. Daru (2017) 25(1):21. doi: 10.1186/s40199-017-0188-7
71. Qiu Z, Dong J, Xue C, Li X, Liu K, Liu B, et al. Liuwei Dihuang Pills alleviate the polycystic ovary syndrome with improved insulin sensitivity through PI3K/Akt signaling pathway. J Ethnopharmacol (2020) 250:111965. doi: 10.1016/j.jep.2019.111965
72. Liu M, Zhu H, Zhu Y, Hu X. Guizhi Fuling Wan reduces autophagy of granulosa cell in rats with polycystic ovary syndrome via restoring the PI3K/AKT/mTOR signaling pathway. J Ethnopharmacol (2021) 270:113821. doi: 10.1016/j.jep.2021.113821
73. Wang F, Xie N, Wu Y, Zhang Q, Zhu Y, Dai M, et al. Association between circadian rhythm disruption and polycystic ovary syndrome. Fertil Steril (2021) 115(3):771–81. doi: 10.1016/j.fertnstert.2020.08.1425
74. Chu W, Li S, Geng X, Wang D, Zhai J, Lu G, et al. Long-term environmental exposure of darkness induces hyperandrogenism in PCOS via melatonin receptor 1A and aromatase reduction. Front Cell Dev Biol (2022) 10:954186. doi: 10.3389/fcell.2022.954186
75. Xie F, Zhang J, Zhai M, Liu Y, Hu H, Yu Z, et al. Melatonin ameliorates ovarian dysfunction by regulating autophagy in PCOS via the PI3K-Akt pathway. Reproduction (2021) 162(1):73–82. doi: 10.1530/REP-20-0643
76. Zheng B, Meng J, Zhu Y, Ding M, Zhang Y, Zhou J. Melatonin enhances SIRT1 to ameliorate mitochondrial membrane damage by activating PDK1/Akt in granulosa cells of PCOS. J Ovarian Res (2021) 14(1):152. doi: 10.1186/s13048-021-00912-y
77. Yi S, Zheng B, Zhu Y, Cai Y, Sun H, Zhou J. Melatonin ameliorates excessive PINK1/Parkin-mediated mitophagy by enhancing SIRT1 expression in granulosa cells of PCOS. Am J Physiol Endocrinol Metab (2020) 319(1):E91–E101. doi: 10.1152/ajpendo.00006.2020
78. Yu K, Wang RX, Li MH, Sun TC, Zhou YW, Li YY, et al. Melatonin reduces androgen production and upregulates heme oxygenase-1 expression in granulosa cells from PCOS patients with hypoestrogenia and hyperandrogenia. Oxid Med Cell Longev (2019) 2019:8218650. doi: 10.1155/2019/8218650
79. Fang L, Li Y, Wang S, Yu Y, Li Y, Guo Y, et al. Melatonin induces progesterone production in human granulosa-lutein cells through upregulation of StAR expression. Aging (Albany NY) (2019) 11(20):9013–24. doi: 10.18632/aging.102367
80. Chen WH, Huang QY, Wang ZY, Zhuang XX, Lin S, Shi QY. Therapeutic potential of exosomes/miRNAs in polycystic ovary syndrome induced by the alteration of circadian rhythms. Front Endocrinol (Lausanne) (2022) 13:918805. doi: 10.3389/fendo.2022.918805
81. Rocha DM, Caldas AP, Oliveira LL, Bressan J, Hermsdorff HH. Saturated fatty acids trigger TLR4-mediated inflammatory response. Atherosclerosis (2016) 244:211–5. doi: 10.1016/j.atherosclerosis.2015.11.015
82. Zusso M, Lunardi V, Franceschini D, Pagetta A, Lo R, Stifani S, et al. Ciprofloxacin and levofloxacin attenuate microglia inflammatory response via TLR4/NF-kB pathway. J Neuroinflammation (2019) 16(1):148. doi: 10.1186/s12974-019-1538-9
83. Xiang P, Chen T, Mou Y, Wu H, Xie P, Lu G, et al. NZ suppresses TLR4/NF-κB signalings and NLRP3 inflammasome activation in LPS-induced RAW264.7 macrophages. Inflammation Res (2015) 64(10):799–808. doi: 10.1007/s00011-015-0863-4
84. Hu M, Zhang Y, Li X, Cui P, Sferruzzi-Perri AN, Brännström M, et al. TLR4-associated IRF-7 and NFκB signaling act as a molecular link between androgen and metformin activities and cytokine synthesis in the PCOS endometrium. J Clin Endocrinol Metab (2021) 106(4):1022–40. doi: 10.1210/clinem/dgaa951
85. Ha LX, Wu YY, Yin T, Yuan YY, Du YD. Effect of TNF-alpha on endometrial glucose transporter-4 expression in patients with polycystic ovary syndrome through nuclear factor-kappa B signaling pathway activation. J Physiol Pharmacol (2021) 72(6):965–73. doi: 10.26402/jpp.2021.6.13
86. Oróstica L, García P, Vera C, García V, Romero C, Vega M. Effect of TNF-α on molecules related to the insulin action in endometrial cells exposed to hyperandrogenic and hyperinsulinic conditions characteristics of polycystic ovary syndrome. Reprod Sci (2018) 25(7):1000–9. doi: 10.1177/1933719117732157
87. Koc O, Ozdemirici S, Acet M, Soyturk U, Aydin S. Nuclear factor-κB expression in the endometrium of normal and overweight women with polycystic ovary syndrome. J Obstet Gynaecol (2017) 37(7):924–30. doi: 10.1080/01443615.2017.1315563
88. Oróstica L, Astorga I, Plaza-Parrochia F, Vera C, García V, Carvajal R, et al. Proinflammatory environment and role of TNF-α in endometrial function of obese women having polycystic ovarian syndrome. Int J Obes (Lond) (2016) 40(11):1715–22. doi: 10.1038/ijo.2016.154
89. Liu Y, Liu H, Li Z, Fan H, Yan X, Liu X, et al. The release of peripheral immune inflammatory cytokines promote an inflammatory cascade in PCOS patients via altering the follicular microenvironment. Front Immunol (2021) 12:685724. doi: 10.3389/fimmu.2021.685724
90. Tan W, Dai F, Yang D, Deng Z, Gu R, Zhao X, et al. MiR-93-5p promotes granulosa cell apoptosis and ferroptosis by the NF-kB signaling pathway in polycystic ovary syndrome. Front Immunol (2022) 13:967151. doi: 10.3389/fimmu.2022.967151
91. Pellegrini L, Foglio E, Pontemezzo E, Germani A, Russo MA, Limana F. HMGB1 and repair: focus on the heart. Pharmacol Ther (2019) 196:160–82. doi: 10.1016/j.pharmthera.2018.12.005
92. Yang H, Wang H, Andersson U. Targeting inflammation driven by HMGB1. Front Immunol (2020) 11:484. doi: 10.3389/fimmu.2020.00484
93. Andersson U, Yang H, Harris H. Extracellular HMGB1 as a therapeutic target in inflammatory diseases. Expert Opin Ther Targets (2018) 22(3):263–77. doi: 10.1080/14728222.2018.1439924
94. Wang HH, Lin M, Xiang G. Serum HMGB1 levels and its association with endothelial dysfunction in patients with polycystic ovary syndrome. Physiol Res (2018) 67(6):911–9. doi: 10.33549/physiolres.933831
95. Ni XR, Sun ZJ, Hu GH, Wang RH. High concentration of insulin promotes apoptosis of primary cultured rat ovarian granulosa cells via its increase in extracellular HMGB1. Reprod Sci (2015) 22(3):271–7. doi: 10.1177/1933719114549852
96. Zhu HL, Chen YQ, Zhang ZF. Downregulation of lncRNA ZFAS1 and upregulation of microRNA-129 repress endocrine disturbance, increase proliferation and inhibit apoptosis of ovarian granulosa cells in polycystic ovarian syndrome by downregulating HMGB1. Genomics (2020) 112(5):3597–608. doi: 10.1016/j.ygeno.2020.04.011
97. Cirillo F, Catellani C, Lazzeroni P, Sartori C, Nicoli A, Amarri S, et al. MiRNAs regulating insulin sensitivity are dysregulated in polycystic ovary syndrome (PCOS) ovaries and are associated with markers of inflammation and insulin sensitivity. Front Endocrinol (Lausanne) (2019) 10:879. doi: 10.3389/fendo.2019.00879
98. Zhang C, Hu J, Wang W, Sun Y, Sun K. HMGB1-induced aberrant autophagy contributes to insulin resistance in granulosa cells in PCOS. FASEB J (2020) 34(7):9563–74. doi: 10.1096/fj.202000605RR
99. Rabadi MM, Xavier S, Vasko R, Kaur K, Goligorksy MS, Ratliff BB. High-mobility group box 1 is a novel deacetylation target of Sirtuin1. Kidney Int (2015) 87(1):95–108. doi: 10.1038/ki.2014.217
100. Andersson U, Tracey KJ, Yang H. Post-translational modification of HMGB1 disulfide bonds in stimulating and inhibiting inflammation. Cells (2021) 10(12):3323. doi: 10.3390/cells10123323
101. Furat Rencber S, Kurnaz Ozbek S, Eraldemır C, Sezer Z, Kum T, Ceylan S, et al. Effect of resveratrol and metformin on ovarian reserve and ultrastructure in PCOS: an experimental study. J Ovarian Res (2018) 11(1):55. doi: 10.1186/s13048-018-0427-7
102. Tao X, Cai L, Chen L, Ge S, Deng X. Effects of metformin and Exenatide on insulin resistance and AMPKα-SIRT1 molecular pathway in PCOS rats. J Ovarian Res (2019) 12:86. doi: 10.1186/s13048-019-0555-8
103. Di Nicuolo F, Castellani R, Ticconi C, Scambia G, Pontecorvi A, Di Simone N. α-lipoic acid and its role on female reproduction. Curr Protein Pept Sci (2021) 22(11):767–74. doi: 10.2174/1389203722666211029102417
104. Yang Y, Yang L, Qi C, Hu G, Wang L, Sun Z, et al. Cryptotanshinone alleviates polycystic ovary syndrome in rats by regulating the HMGB1/TLR4/NF−κB signaling pathway. Mol Med Rep (2020) 22(5):3851–61. doi: 10.3892/mmr.2020.11469
105. Li Y, Huang H, Liu B, Zhang Y, Pan X, Yu XY, et al. Inflammasomes as therapeutic targets in human diseases. Signal Transduct Target Ther (2021) 6(1):247. doi: 10.1038/s41392-021-00650-z
106. Chan AH, Schroder K. Inflammasome signaling and regulation of interleukin-1 family cytokines. J Exp Med (2020) 217(1):e20190314. doi: 10.1084/jem.20190314
107. Wang D, Weng Y, Zhang Y, Wang R, Wang T, Zhou J, et al. Exposure to hyperandrogen drives ovarian dysfunction and fibrosis by activating the NLRP3 inflammasome in mice. Sci Total Environ (2020) 745:141049. doi: 10.1016/j.scitotenv.2020.141049
108. Lai Y, Ye Z, Mu L, Zhang Y, Long X, Zhang C, et al. Elevated levels of follicular fatty acids induce ovarian inflammation via ERK1/2 and inflammasome activation in PCOS. J Clin Endocrinol Metab (2022) 107(8):2307–17. doi: 10.1210/clinem/dgac281
109. Rostamtabar M, Esmaeilzadeh S, Karkhah A, Amiri M, Rahmani A, Bakouei F, et al. Elevated expression of IL-18 but not IL-1β gene is associated with NALP3 and AIM2 inflammasome in Polycystic Ovary Syndrome. Gene (2020) 731:144352. doi: 10.1016/j.gene.2020.144352
110. Olaniyi KS, Areloegbe SE, Oyeleke MB. Acetate restores hypothalamic-adipose kisspeptin status in a rat model of PCOS by suppression of NLRP3 immunoreactivity. Endocrine (2022) 78(3):628–40. doi: 10.1007/s12020-022-03191-9
111. Li Y, Yao N, Gao Y, Wang Y, Bai L, Xu J, et al. MiR-1224-5p attenuates polycystic ovary syndrome through inhibiting NOD-like receptor protein 3 inflammasome activation via targeting Forkhead box O 1. Bioengineered (2021) 12(1):8555–69. doi: 10.1080/21655979.2021.1987125
112. Zhou F, Li C, Zhang SY. NLRP3 inflammasome: a new therapeutic target for high-risk reproductive disorders? Chin Med J (Engl) (2020) 134(1):20–7. doi: 10.1097/CM9.0000000000001214
113. Guo QJ, Shan J, Xu YF, Hu YY, Huo CL, Song JY, et al. Pioglitazone metformin complex improves polycystic ovary syndrome comorbid psychological distress via inhibiting NLRP3 inflammasome activation: A prospective clinical study. Mediators Inflamm (2020) 2020:3050487. doi: 10.1155/2020/3050487
114. Cai Z, He S, Liu R, Zhou L, Zhao L. Plumbagin rescues the granulosa cell’s pyroptosis by reducing WTAP-mediated N6-methylation in polycystic ovary syndrome. J Ovarian Res (2022) 15(1):126. doi: 10.1186/s13048-022-01058-1
115. Wang C, Li Z, Liu Y, Yuan L. Exosomes in atherosclerosis: performers, bystanders, biomarkers, and therapeutic targets. Theranostics (2021) 11(8):3996–4010. doi: 10.7150/thno.56035
116. Li H, Huang X, Chang X, Yao J, He Q, Shen Z, et al. S100-A9 protein in exosomes derived from follicular fluid promotes inflammation via activation of NF-κB pathway in polycystic ovary syndrome. J Cell Mol Med (2020) 24(1):114–25. doi: 10.1111/jcmm.14642
117. Xie Q, Xiong X, Xiao N, He K, Chen M, Peng J, et al. Mesenchymal stem cells alleviate DHEA-induced polycystic ovary syndrome (PCOS) by inhibiting inflammation in mice. Stem Cells Int (2019) 2019:9782373. doi: 10.1155/2019/9782373
118. Cao M, Zhao Y, Chen T, Zhao Z, Zhang B, Yuan C, et al. Adipose mesenchymal stem cell-derived exosomal microRNAs ameliorate polycystic ovary syndrome by protecting against metabolic disturbances. Biomaterials (2022) 288:121739. doi: 10.1016/j.biomaterials.2022.121739
119. Esfandyari S, Chugh RM, Park HS, Hobeika E, Ulin M, Al-Hendy A. Mesenchymal stem cells as a bio organ for treatment of female infertility. Cells (2020) 9(10):2253. doi: 10.3390/cells9102253
120. Noonin C, Thongboonkerd V. Exosome-inflammasome crosstalk and their roles in inflammatory responses. Theranostics (2021) 11(9):4436–51. doi: 10.7150/thno.54004
121. Zhou W, Zhang T, Lian Y, Zhang W, Yang M, Li Y, et al. Exosomal lncRNA and mRNA profiles in polycystic ovary syndrome: bioinformatic analysis reveals disease-related networks. Reprod BioMed Online (2022) 44(5):777–90. doi: 10.1016/j.rbmo.2022.01.007
122. Zhang F, Li SP, Zhang T, Yu B, Zhang J, Ding HG, et al. High Throughput Micrornas Sequencing Profile of Serum Exosomes in Women with and without Polycystic Ovarian Syndrome. PeerJ (2021) 9:e10998. doi: 10.7717/peerj.10998
123. Giampaolino P, Foreste V, Di Filippo C, Gallo A, Mercorio A, Serafino P, et al. Microbiome and PCOS: state-of-art and future aspects. Int J Mol Sci (2021) 22(4):2048. doi: 10.3390/ijms22042048
124. Qi X, Yun C, Sun L, Xia J, Wu Q, Wang Y, et al. Gut microbiota-bile acid-interleukin-22 axis orchestrates polycystic ovary syndrome. Nat Med (2019) 25(8):1225–33. doi: 10.1038/s41591-019-0509-0
125. He FF, Li YM. Role of gut microbiota in the development of insulin resistance and the mechanism underlying polycystic ovary syndrome: a review. J Ovarian Res (2020) 13(1):73. doi: 10.1186/s13048-020-00670-3
126. Wang Y, Xiao H, Liu Y, Tong Q, Yu Y, Qi B, et al. Effects of Bu Shen Hua Zhuo formula on the LPS/TLR4 pathway and gut microbiota in rats with letrozole-induced polycystic ovary syndrome. Front Endocrinol (Lausanne) (2022) 13:891297. doi: 10.3389/fendo.2022.891297
127. Chang ZP, Deng GF, Shao YY, Xu D, Zhao YN, Sun YF, et al. Shaoyao-gancao decoction ameliorates the inflammation state in polycystic ovary syndrome rats via remodeling gut microbiota and suppressing the TLR4/NF-κB pathway. Front Pharmacol (2021) 12:670054. doi: 10.3389/fphar.2021.670054
128. Silva MSB, Giacobini P. Don’t trust your gut: when gut microbiota disrupt fertility. Cell Metab (2019) 30(4):616–8. doi: 10.1016/j.cmet.2019.09.005
129. Rahmani E, Jamilian M, Samimi M, Zarezade Mehrizi M, Aghadavod E, Akbari E, et al. The effects of coenzyme Q10 supplementation on gene expression related to insulin, lipid and inflammation in patients with polycystic ovary syndrome. Gynecol Endocrinol (2018) 34(3):217–22. doi: 10.1080/09513590.2017.1381680
130. Jamilian M, Shojaei A, Samimi M, Afshar Ebrahimi F, Aghadavod E, Karamali M, et al. The effects of omega-3 and vitamin E co-supplementation on parameters of mental health and gene expression related to insulin and inflammation in subjects with polycystic ovary syndrome. J Affect Disord (2018) 229:41–7. doi: 10.1016/j.jad.2017.12.049
131. Nasri K, Hantoushzadeh S, Aghadavod E, Taghizadeh M, Asemi Z. The effects of omega-3 fatty acids supplementation on gene expression involved in the insulin and lipid signaling pathway in patients with polycystic ovary syndrome. Horm Metab Res (2017) 49(6):446–51. doi: 10.1055/s-0042-122782
132. Baird L, Yamamoto M. The molecular mechanisms regulating the KEAP1-NRF2 pathway. Mol Cell Biol (2020) 40(13):e00099–20. doi: 10.1128/MCB.00099-20
133. Zhang H, Gao Z, Zhang Y, Wang H, Li Y. MiR-873-5p regulated LPS-induced oxidative stress via targeting heme oxygenase-1 (HO-1) in KGN cells. RSC Adv (2018) 8(68):39098–105. doi: 10.1039/c8ra06697c
134. Wang Y, Zeng Z, Zhao S, Tang L, Yan J, Li N, et al. Humanin alleviates insulin resistance in polycystic ovary syndrome: A human and rat model-based study. Endocrinology (2021) 162(8):bqab056. doi: 10.1210/endocr/bqab056
135. Wang Y, Li N, Zeng Z, Tang L, Zhao S, Zhou F, et al. Humanin regulates oxidative stress in the ovaries of polycystic ovary syndrome patients via the Keap1/Nrf2 pathway. Mol Hum Reprod (2021) 27(2):gaaa081. doi: 10.1093/molehr/gaaa081
136. Ahmed SMU, Luo L, Namani A, Wang XJ, Tang X. Nrf2 signaling pathway: Pivotal roles in inflammation. Biochim Biophys Acta Mol Basis Dis (2017) 1863(2):585–97. doi: 10.1016/j.bbadis.2016.11.005
137. Zhang J, Wang X, Vikash V, Ye Q, Wu D, Liu Y, et al. ROS and ROS-mediated cellular signaling. Oxid Med Cell Longev (2016) 2016:4350965. doi: 10.1155/2016/4350965
138. Matzinger M, Fischhuber K, Pölöske D, Mechtler K, Heiss EH. AMPK leads to phosphorylation of the transcription factor Nrf2, tuning transactivation of selected target genes. Redox Biol (2020) 29:101393. doi: 10.1016/j.redox.2019.101393
139. Wang X, Chen X, Zhou W, Men H, Bao T, Sun Y, et al. Ferroptosis is essential for diabetic cardiomyopathy and is prevented by sulforaphane via AMPK/NRF2 pathways. Acta Pharm Sin B (2022) 12(2):708–22. doi: 10.1016/j.apsb.2021.10.005
140. Su X, Jiang X, Meng L, Dong X, Shen Y, Xin Y. Anticancer activity of sulforaphane: the epigenetic mechanisms and the nrf2 signaling pathway. Oxid Med Cell Longev (2018) 2018:5438179. doi: 10.1155/2018/5438179
141. Bose C, Alves I, Singh P, Palade PT, Carvalho E, Børsheim E, et al. Sulforaphane prevents age-associated cardiac and muscular dysfunction through Nrf2 signaling. Aging Cell (2020) 19(11):e13261. doi: 10.1111/acel.13261
142. Lei P, Tian S, Teng C, Huang L, Liu X, Wang J, et al. Sulforaphane improves lipid metabolism by enhancing mitochondrial function and biogenesis in vivo and in vitro. Mol Nutr Food Res (2019) 63(4):e1800795. doi: 10.1002/mnfr.201800795
143. Pastorek M, Simko V, Takacova M, Barathova M, Bartosova M, Hunakova L, et al. Sulforaphane reduces molecular response to hypoxia in ovarian tumor cells independently of their resistance to chemotherapy. Int J Oncol (2015) 47(1):51–60. doi: 10.3892/ijo.2015.2987
144. Taheri M, Hayati Roudbari N, Amidi F, Parivar K. The protective effect of sulforaphane against oxidative stress in granulosa cells of patients with polycystic ovary syndrome (PCOS) through activation of AMPK/AKT/NRF2 signaling pathway. Reprod Biol (2021) 21(4):100563. doi: 10.1016/j.repbio.2021.100563
145. Ji R, Jia FY, Chen X, Wang ZH, Jin WY, Yang J. Salidroside alleviates oxidative stress and apoptosis via AMPK/Nrf2 pathway in DHT-induced human granulosa cell line KGN. Arch Biochem Biophys (2022) 715:109094. doi: 10.1016/j.abb.2021.109094
146. Bi Y, Lei X, Chai N, Linghu E. NOX4: a potential therapeutic target for pancreatic cancer and its mechanism. J Transl Med (2021) 19(1):515. doi: 10.1186/s12967-021-03182-w
147. Yao Q, Zou X, Liu S, Wu H, Shen Q, Kang J. Oxidative stress as a contributor to insulin resistance in the skeletal muscles of mice with polycystic ovary syndrome. Int J Mol Sci (2022) 23(19):11384. doi: 10.3390/ijms231911384
148. Li Y, Xu J, Li L, Bai L, Wang Y, Zhang J, et al. Inhibition of Nicotinamide adenine dinucleotide phosphate oxidase 4 attenuates cell apoptosis and oxidative stress in a rat model of polycystic ovary syndrome through the activation of Nrf-2/HO-1 signaling pathway. Mol Cell Endocrinol (2022) 550:111645. doi: 10.1016/j.mce.2022.111645
149. Smyrnias I, Zhang X, Zhang M, Murray TV, Brandes RP, Schröder K, et al. Nicotinamide adenine dinucleotide phosphate oxidase-4-dependent upregulation of nuclear factor erythroid-derived 2-like 2 protects the heart during chronic pressure overload. Hypertension (2015) 65(3):547–53. doi: 10.1161/HYPERTENSIONAHA.114.04208
150. Abrahao TB, Griendling KK. Nuclear factor (erythroid-derived 2)-like 2, the brake in oxidative stress that nicotinamide adenine dinucleotide phosphate-oxidase-4 needs to protect the heart. Hypertension (2015) 65(3):499–501. doi: 10.1161/HYPERTENSIONAHA.114.04347
151. Mihaylova MM, Shaw RJ. The AMPK signalling pathway coordinates cell growth, autophagy and metabolism. Nat Cell Biol (2011) 13(9):1016–23. doi: 10.1038/ncb2329
152. Hardie DG, Schaffer BE, Brunet A. AMPK: an energy-sensing pathway with multiple inputs and outputs. Trends Cell Biol (2016) 26(3):190–201. doi: 10.1016/j.tcb.2015.10.013
153. Froment P, Plotton I, Giulivi C, Fabre S, Khoueiry R, Mourad NI, et al. At the crossroads of fertility and metabolism: the importance of AMPK-dependent signaling in female infertility associated with hyperandrogenism. Hum Reprod (2022) 37(6):1207–28. doi: 10.1093/humrep/deac067
154. Zhang X, Zhang W, Wang Z, Zheng N, Yuan F, Li B, et al. Enhanced glycolysis in granulosa cells promotes the activation of primordial follicles through mTOR signaling. Cell Death Dis (2022) 13(1):87. doi: 10.1038/s41419-022-04541-1
155. McDonnell T, Cussen L, McIlroy M, O’Reilly MW. Characterizing skeletal muscle dysfunction in women with polycystic ovary syndrome. Ther Adv Endocrinol Metab (2022) 13:20420188221113140. doi: 10.1177/20420188221113140
156. Mićić B, Teofilović A, Djordjevic A, Veličković N, Macut D, Vojnović Milutinović D. AMPK activation is important for the preservation of insulin sensitivity in visceral, but not in subcutaneous adipose tissue of postnatally overfed rat model of polycystic ovary syndrome. Int J Mol Sci (2022) 23(16):8942. doi: 10.3390/ijms23168942
157. Parker J, O’Brien C, Hawrelak J, Gersh FL. Polycystic ovary syndrome: an evolutionary adaptation to lifestyle and the environment. Int J Environ Res Public Health (2022) 19(3):1336. doi: 10.3390/ijerph19031336
158. Estienne A, Bongrani A, Ramé C, Kurowska P, Błaszczyk K, Rak A, et al. Energy sensors and reproductive hypothalamo-pituitary ovarian axis (HPO) in female mammals: Role of mTOR (mammalian target of rapamycin), AMPK (AMP-activated protein kinase) and SIRT1 (Sirtuin 1). Mol Cell Endocrinol (2021) 521:111113. doi: 10.1016/j.mce.2020.111113
159. Wu M, Zhang J, Gu R, Dai F, Yang D, Zheng Y, et al. The role of Sirtuin 1 in the pathophysiology of polycystic ovary syndrome. Eur J Med Res (2022) 27(1):158. doi: 10.1186/s40001-022-00746-4
160. Di Emidio G, Santini SJ, D’Alessandro AM, Vetuschi A, Sferra R, Artini PG, et al. SIRT1 participates in the response to methylglyoxal-dependent glycative stress in mouse oocytes and ovary. Biochim Biophys Acta Mol Basis Dis (2019) 1865(6):1389–401. doi: 10.1016/j.bbadis.2019.02.011
161. Zhang Q, Ren J, Wang F, Pan M, Cui L, Li M, et al. Mitochondrial and glucose metabolic dysfunctions in granulosa cells induce impaired oocytes of polycystic ovary syndrome through Sirtuin 3. Free Radic Biol Med (2022) 187:1–16. doi: 10.1016/j.freeradbiomed.2022.05.010
162. Pang X, Cheng J, Wu T, Sun L. SIRT3 ameliorates polycystic ovary syndrome through FOXO1/PGC-1α signaling pathway. Endocrine (2023) 80(1):201–11. doi: 10.1007/s12020-022-03262-x
163. Li X, Qi J, Zhu Q, He Y, Wang Y, Lu Y, et al. The role of androgen in autophagy of granulosa cells from PCOS. Gynecol Endocrinol (2019) 35(8):669–72. doi: 10.1080/09513590.2018.1540567
164. Li T, Dong G, Kang Y, Zhang M, Sheng X, Wang Z, et al. Increased homocysteine regulated by androgen activates autophagy by suppressing the mammalian target of rapamycin pathway in the granulosa cells of polycystic ovary syndrome mice. Bioengineered (2022) 13(4):10875–88. doi: 10.1080/21655979.2022.2066608
165. Koh A, Mannerås-Holm L, Yunn NO, Nilsson PM, Ryu SH, Molinaro A, et al. Microbial Imidazole Propionate Affects Responses to Metformin through p38γ-Dependent Inhibitory AMPK Phosphorylation. Cell Metab (2020) 32(4):643–653.e4. doi: 10.1016/j.cmet.2020.07.012
166. Chen Z, Wei H, Zhao X, Xin X, Peng L, Ning Y, et al. Metformin treatment alleviates polycystic ovary syndrome by decreasing the expression of MMP-2 and MMP-9 via H19/miR-29b-3p and AKT/mTOR/autophagy signaling pathways. J Cell Physiol (2019) 234(11):19964–76. doi: 10.1002/jcp.28594
167. Siamashvili M, Davis SN. Update on the effects of GLP-1 receptor agonists for the treatment of polycystic ovary syndrome. Expert Rev Clin Pharmacol (2021) 14(9):1081–9. doi: 10.1080/17512433.2021.1933433
168. Cabrera-Cruz H, Oróstica L, Plaza-Parrochia F, Torres-Pinto I, Romero C, Vega M. The insulin-sensitizing mechanism of myo-inositol is associated with AMPK activation and GLUT-4 expression in human endometrial cells exposed to a PCOS environment. Am J Physiol Endocrinol Metab (2020) 318(2):E237–48. doi: 10.1152/ajpendo.00162.2019
169. Tabatabaie M, Amiri S, Golestan Jahromi M, Sene AA, Zandieh Z, Mehdizadeh M, et al. The effect of Myo-Inositol supplement on molecular regulation of folliculogenesis, steroidogenesis, and assisted reproductive technique outcomes in patients with polycystic ovarian syndrome. Mol Biol Rep (2022) 49(2):875–84. doi: 10.1007/s11033-021-06833-9
170. Gao L, Cao JT, Liang Y, Zhao YC, Lin XH, Li XC, et al. Calcitriol attenuates cardiac remodeling and dysfunction in a murine model of polycystic ovary syndrome. Endocrine (2016) 52(2):363–73. doi: 10.1007/s12020-015-0797-1
171. Nejabati HR, Samadi N, Shahnazi V, Mihanfar A, Fattahi A, Latifi Z, et al. Nicotinamide and its metabolite N1-Methylnicotinamide alleviate endocrine and metabolic abnormalities in adipose and ovarian tissues in rat model of Polycystic Ovary Syndrome. Chem Biol Interact (2020) 324:109093. doi: 10.1016/j.cbi.2020.109093
172. Wang Y, Yang Q, Wang H, Zhu J, Cong L, Li H, et al. NAD+ deficiency and mitochondrial dysfunction in granulosa cells of women with polycystic ovary syndrome‡. Biol Reprod (2021) 105(2):371–80. doi: 10.1093/biolre/ioab078
173. Nejabati HR, Schmeisser K, Shahnazi V, Samimifar D, Faridvand Y, Bahrami-Asl Z, et al. N1-methylnicotinamide: an anti-ovarian aging hormetin? Ageing Res Rev (2020) 62:101131. doi: 10.1016/j.arr.2020.101131
174. Chen T, Jia F, Yu Y, Zhang W, Wang C, Zhu S, et al. Potential role of quercetin in polycystic ovary syndrome and its complications: A review. Molecules (2022) 27(14):4476. doi: 10.3390/molecules27144476
175. Ma C, Xiang Q, Song G, Wang X. Quercetin and polycystic ovary syndrome. Front Pharmacol (2022) 13:1006678. doi: 10.3389/fphar.2022.1006678
176. Mihanfar A, Nouri M, Roshangar L, Khadem-Ansari MH. Ameliorative effects of fisetin in letrozole-induced rat model of polycystic ovary syndrome. J Steroid Biochem Mol Biol (2021) 213:105954. doi: 10.1016/j.jsbmb.2021.105954
177. Wang W, Zheng J, Cui N, Jiang L, Zhou H, Zhang D, et al. Baicalin ameliorates polycystic ovary syndrome through AMP-activated protein kinase. J Ovarian Res (2019) 12(1):109. doi: 10.1186/s13048-019-0585-2
178. Chen J, Wang L, Yuan M. Update on the roles of rice MAPK cascades. Int J Mol Sci (2021) 22(4):1679. doi: 10.3390/ijms22041679
179. Liang YJ, Yang WX. Kinesins in MAPK cascade: How kinesin motors are involved in the MAPK pathway? Gene (2019) 684:1–9. doi: 10.1016/j.gene.2018.10.042
180. Liu Y, Li Z, Wang Y, Cai Q, Liu H, Xu C, et al. IL-15 participates in the pathogenesis of polycystic ovary syndrome by affecting the activity of granulosa cells. Front Endocrinol (Lausanne) (2022) 13:787876. doi: 10.3389/fendo.2022.787876
181. Peng Y, Yang H, Song J, Feng D, Na Z, Jiang H, et al. Elevated serum leptin levels as a predictive marker for polycystic ovary syndrome. Front Endocrinol (Lausanne) (2022) 13:845165. doi: 10.3389/fendo.2022.845165
182. de Medeiros SF, Rodgers RJ, Norman RJ. Adipocyte and steroidogenic cell cross-talk in polycystic ovary syndrome. Hum Reprod Update (2021) 27(4):771–96. doi: 10.1093/humupd/dmab004
183. Vilariño-García T, Pérez-Pérez A, Santamaría-López E, Prados N, Fernández-Sánchez M, Sánchez-Margalet V. Sam68 mediates leptin signaling and action in human granulosa cells: possible role in leptin resistance in PCOS. Endocr Connect (2020) 9(6):479–88. doi: 10.1530/EC-20-0062
184. Vilariño-García T, Guadix P, Dorado-Silva M, Sánchez-Martín P, Pérez-Pérez A, Sánchez-Margalet V. Decreased expression of sam68 is associated with insulin resistance in granulosa cells from PCOS patients. Cells (2022) 11(18):2821. doi: 10.3390/cells11182821
185. Kobayashi M, Yoshino O, Nakashima A, Ito M, Nishio K, Ono Y, et al. Inhibition of autophagy in theca cells induces CYP17A1 and PAI-1 expression via ROS/p38 and JNK signalling during the development of polycystic ovary syndrome. Mol Cell Endocrinol (2020) 508:110792. doi: 10.1016/j.mce.2020.110792
186. Qin Y, Li T, Zhao H, Mao Z, Ding C, Kang Y. Integrated transcriptomic and epigenetic study of PCOS: impact of map3k1 and map1lc3a promoter methylation on autophagy. Front Genet (2021) 12:620241. doi: 10.3389/fgene.2021.620241
187. Huang JC, Duan CC, Jin S, Sheng CB, Wang YS, Yue ZP, et al. HB-EGF induces mitochondrial dysfunction via estrogen hypersecretion in granulosa cells dependent on cAMP-PKA-JNK/ERK-Ca2+-FOXO1 pathway. Int J Biol Sci (2022) 18(5):2047–59. doi: 10.7150/ijbs.69343
188. Lan CW, Chen MJ, Tai KY, Yu DC, Yang YC, Jan PS, et al. Functional microarray analysis of differentially expressed genes in granulosa cells from women with polycystic ovary syndrome related to MAPK/ERK signaling. Sci Rep (2015) 5:14994. doi: 10.1038/srep14994
189. Cheng JC, Han X, Meng Q, Guo Y, Liu B, Song T, et al. HB-EGF upregulates StAR expression and stimulates progesterone production through ERK1/2 signaling in human granulosa-lutein cells. Cell Commun Signal (2022) 20(1):166. doi: 10.1186/s12964-022-00983-4
190. Qi J, Li J, Wang Y, Wang W, Zhu Q, He Y, et al. Novel role of CXCL14 in modulating STAR expression in luteinized granulosa cells: implication for progesterone synthesis in PCOS patients. Transl Res (2021) 230:55–67. doi: 10.1016/j.trsl.2020.10.009
191. Sucquart IE, Nagarkar R, Edwards MC, Rodriguez Paris V, Aflatounian A, Bertoldo MJ, et al. Neurokinin 3 receptor antagonism ameliorates key metabolic features in a hyperandrogenic PCOS mouse model. Endocrinology (2021) 162(5):bqab020. doi: 10.1210/endocr/bqab020
192. Zhang L, Fernando T, Liu Y, Liu Y, Zhu X, Li M, et al. Neurokinin 3 receptor antagonist-induced adipocyte activation improves obesity and metabolism in PCOS-like mice. Life Sci (2022) 310:121078. doi: 10.1016/j.lfs.2022.121078
193. Guo F, Fernando T, Zhu X, Shi Y. The overexpression of neurokinin B-neurokinin 3 receptor system exerts direct effects on the ovary under PCOS-like conditions to interfere with mitochondrial function. Am J Reprod Immunol (2023) 89(3):e13663. doi: 10.1111/aji.13663
194. Jin J, Ma Y, Tong X, Yang W, Dai Y, Pan Y, et al. Metformin inhibits testosterone-induced endoplasmic reticulum stress in ovarian granulosa cells via inactivation of p38 MAPK. Hum Reprod (2020) 35(5):1145–58. doi: 10.1093/humrep/deaa077
195. Jiang XL, Tai H, Xiao XS, Zhang SY, Cui SC, Qi SB, et al. Cangfudaotan decoction inhibits mitochondria-dependent apoptosis of granulosa cells in rats with polycystic ovarian syndrome. Front Endocrinol (Lausanne) (2022) 13:962154. doi: 10.3389/fendo.2022.962154
196. Lerner A, Kewada D, Ahmed A, Hardy K, Christian M, Franks S. Androgen reduces mitochondrial respiration in mouse brown adipocytes: A model for disordered energy balance in polycystic ovary syndrome. Int J Mol Sci (2020) 22(1):243. doi: 10.3390/ijms22010243
197. Sanchez-Garrido MA, Tena-Sempere M. Metabolic dysfunction in polycystic ovary syndrome: Pathogenic role of androgen excess and potential therapeutic strategies. Mol Metab (2020) 35:100937. doi: 10.1016/j.molmet.2020.01.001
198. Yao L, Wang Q, Zhang R, Wang X, Liu Y, Di F, et al. Brown adipose transplantation improves polycystic ovary syndrome-involved metabolome remodeling. Front Endocrinol (Lausanne) (2021) 12:747944. doi: 10.3389/fendo.2021.747944
199. Cai G, Ma X, Chen B, Huang Y, Liu S, Yang H, et al. MicroRNA-145 negatively regulates cell proliferation through targeting IRS1 in isolated ovarian granulosa cells from patients with polycystic ovary syndrome. Reprod Sci (2017) 24(6):902–10. doi: 10.1177/1933719116673197
200. Fragoulis GE, McInnes IB, Siebert S. JAK-inhibitors. New players in the field of immune-mediated diseases, beyond rheumatoid arthritis. Rheumatol (Oxford) (2019) 58(Suppl 1):i43–54. doi: 10.1093/rheumatology/key276
201. Lokau J, Garbers C. Activating mutations of the gp130/JAK/STAT pathway in human diseases. Adv Protein Chem Struct Biol (2019) 116:283–309. doi: 10.1016/bs.apcsb.2018.11.007
202. Hatzirodos N, Irving-Rodgers HF, Hummitzsch K, Harland ML, Morris SE, Rodgers RJ. Transcriptome profiling of granulosa cells of bovine ovarian follicles during growth from small to large antral sizes. BMC Genomics (2014) 15:24. doi: 10.1186/1471-2164-15-24
203. Ho CH, Chang CM, Li HY, Shen HY, Lieu FK, Wang PSG. Dysregulated immunological and metabolic functions discovered by a polygenic integrative analysis for PCOS. Reprod BioMed Online (2020) 40(1):160–7. doi: 10.1016/j.rbmo.2019.09.011
204. Maliqueo M, Sundström Poromaa I, Vanky E, Fornes R, Benrick A, Åkerud H, et al. Placental STAT3 signaling is activated in women with polycystic ovary syndrome. Hum Reprod (2015) 30(3):692–700. doi: 10.1093/humrep/deu351
205. Zhuang Z, Pan X, Zhao K, Gao W, Liu J, Deng T, et al. The effect of interleukin-6 (IL-6), interleukin-11 (IL-11), signal transducer and activator of transcription 3 (STAT3), and AKT signaling on adipocyte proliferation in a rat model of polycystic ovary syndrome. Med Sci Monit (2019) 25:7218–27. doi: 10.12659/MSM.916385
206. Borthakur A, Prabhu Y D, Valsala Gopalakrishnan A. Role of IL-6 signalling in Polycystic Ovarian Syndrome associated inflammation. J Reprod Immunol (2020) 141:103155. doi: 10.1016/j.jri.2020.103155
207. Huang YH, Dong LP, Cui YG, Lu HY. MiR-520h inhibits viability and facilitates apoptosis of KGN cells through modulating IL6R and the JAK/STAT pathway. Reprod Biol (2022) 22(1):100607. doi: 10.1016/j.repbio.2022.100607
208. Baldini C, Moriconi FR, Galimberti S, Libby P, De Caterina R. The JAK-STAT pathway: an emerging target for cardiovascular disease in rheumatoid arthritis and myeloproliferative neoplasms. Eur Heart J (2021) 42(42):4389–400. doi: 10.1093/eurheartj/ehab447
209. Shao S, Wang H, Shao W, Liu N. miR-199a-5p stimulates ovarian granulosa cell apoptosis in polycystic ovary syndrome. J Mol Endocrinol (2020) 65(4):187–201. doi: 10.1530/JME-20-0077
210. Zhou Y, Lv L, Liu Q, Song J. Total flavonoids extracted from Nervilia Fordii function in polycystic ovary syndrome through IL-6 mediated JAK2/STAT3 signaling pathway. Biosci Rep (2019) 39(1):BSR20181380. doi: 10.1042/BSR20181380
211. Gao Z, Wang G, Ma X, Tan H, Zhang C, Yin X, et al. Troxerutin attenuates insulin resistance via pancreatic IL-22/JAK1/STAT3 signaling activation in dihydrotestosterone-induced polycystic ovary syndrome rats. Am J Physiol Endocrinol Metab (2022) 323(5):E405–17. doi: 10.1152/ajpendo.00150.2022
212. Ma S, Meng Z, Chen R, Guan KL. The hippo pathway: biology and pathophysiology. Annu Rev Biochem (2019) 88:577–604. doi: 10.1146/annurev-biochem-013118-111829
213. Hsueh AJW, Kawamura K, Cheng Y, Fauser BCJM. Intraovarian control of early folliculogenesis. Endocr Rev (2015) 36(1):1–24. doi: 10.1210/er.2014-1020
214. Driskill JH, Pan D. The hippo pathway in liver homeostasis and pathophysiology. Annu Rev Pathol (2021) 16:299–322. doi: 10.1146/annurev-pathol-030420-105050
215. Wang D, He J, Huang B, Liu S, Zhu H, Xu T. Emerging role of the Hippo pathway in autophagy. Cell Death Dis (2020) 11(10):880. doi: 10.1038/s41419-020-03069-6
216. Clark KL, George JW, Przygrodzka E, Plewes MR, Hua G, Wang C, et al. Hippo signaling in the ovary: emerging roles in development, fertility, and disease. Endocr Rev (2022) 43(6):1074–96. doi: 10.1210/endrev/bnac013
217. Lunding SA, Andersen AN, Hardardottir L, Olesen H, Kristensen SG, Andersen CY, et al. Hippo signaling, actin polymerization, and follicle activation in fragmented human ovarian cortex. Mol Reprod Dev (2020) 87(6):711–9. doi: 10.1002/mrd.23353
218. Kinnear HM, Tomaszewski CE, Chang FL, Moravek MB, Xu M, Padmanabhan V, et al. The ovarian stroma as a new frontier. Reproduction (2020) 160(3):R25–39. doi: 10.1530/REP-19-0501
219. Chen H, Cheng S, Xiong W, Tan X. The lncRNA-miRNA-mRNA ceRNA network in mural granulosa cells of patients with polycystic ovary syndrome: an analysis of Gene Expression Omnibus data. Ann Transl Med (2021) 9(14):1156. doi: 10.21037/atm-21-2696
220. Maas K, Mirabal S, Penzias A, Sweetnam PM, Eggan KC, Sakkas D. Hippo signaling in the ovary and polycystic ovarian syndrome. J Assist Reprod Genet (2018) 35(10):1763–71. doi: 10.1007/s10815-018-1235-0
221. Li T, Zhao H, Zhao X, Zhang B, Cui L, Shi Y, et al. Identification of YAP1 as a novel susceptibility gene for polycystic ovary syndrome. J Med Genet (2012) 49(4):254–7. doi: 10.1136/jmedgenet-2011-100727
222. Zhang Y, Ho K, Keaton JM, Hartzel DN, Day F, Justice AE, et al. A genome-wide association study of polycystic ovary syndrome identified from electronic health records. Am J Obstet Gynecol (2020) 223(4):559.e1–559.e21. doi: 10.1016/j.ajog.2020.04.004
223. Lidaka L, Bekere L, Lazdane G, Lazovska M, Dzivite-Krisane I, Gailite L. Role of single nucleotide variants in the YAP1 gene in adolescents with polycystic ovary syndrome. Biomedicines (2022) 10(7):1688. doi: 10.3390/biomedicines10071688
224. Jiang LL, Xie JK, Cui JQ, Wei D, Yin BL, Zhang YN, et al. Promoter methylation of yes-associated protein (YAP1) gene in polycystic ovary syndrome. Med (Baltimore) (2017) 96(2):e5768. doi: 10.1097/MD.0000000000005768
225. Ji SY, Liu XM, Li BT, Zhang YL, Liu HB, Zhang YC, et al. The polycystic ovary syndrome-associated gene Yap1 is regulated by gonadotropins and sex steroid hormones in hyperandrogenism-induced oligo-ovulation in mouse. Mol Hum Reprod (2017) 23(10):698–707. doi: 10.1093/molehr/gax046
226. Sun T, Diaz FJ. Ovulatory signals alter granulosa cell behavior through YAP1 signaling. Reprod Biol Endocrinol (2019) 17(1):113. doi: 10.1186/s12958-019-0552-1
227. Wang H, Cai H, Wang X, Zhang M, Liu B, Chen Z, et al. HDAC3 maintains oocyte meiosis arrest by repressing amphiregulin expression before the LH surge. Nat Commun (2019) 10(1):5719. doi: 10.1038/s41467-019-13671-8
228. Liu Y, Zhong Y, Shen X, Guo X, Wu R, Yang T, et al. Luteinizing hormone stimulates the expression of amphiregulin in human theca cells. J Ovarian Res (2022) 15(1):129. doi: 10.1186/s13048-022-01062-5
229. Kawashima I, Kawamura K. Regulation of follicle growth through hormonal factors and mechanical cues mediated by Hippo signaling pathway. Syst Biol Reprod Med (2018) 64(1):3–11. doi: 10.1080/19396368.2017.1411990
230. Santinon G, Pocaterra A, Dupont S. Control of YAP/TAZ activity by metabolic and nutrient-sensing pathways. Trends Cell Biol (2016) 26(4):289–99. doi: 10.1016/j.tcb.2015.11.004
231. Koo JH, Guan KL. Interplay between YAP/TAZ and metabolism. Cell Metab (2018) 28(2):196–206. doi: 10.1016/j.cmet.2018.07.010
232. Seow KM, Chang YW, Chen KH, Juan CC, Huang CY, Lin LT, et al. Molecular mechanisms of laparoscopic ovarian drilling and its therapeutic effects in polycystic ovary syndrome. Int J Mol Sci (2020) 21(21):8147. doi: 10.3390/ijms21218147
233. Parsons MJ, Tammela T, Dow LE. WNT as a driver and dependency in cancer. Cancer Discovery (2021) 11(10):2413–29. doi: 10.1158/2159-8290.CD-21-0190
234. Sharma M, Pruitt K. Wnt pathway: an integral hub for developmental and oncogenic signaling networks. Int J Mol Sci (2020) 21(21):8018. doi: 10.3390/ijms21218018
235. Ghosh N, Hossain U, Mandal A, Sil PC. The Wnt signaling pathway: a potential therapeutic target against cancer. Ann N Y Acad Sci (2019) 1443(1):54–74. doi: 10.1111/nyas.14027
236. Gajos-Michniewicz A, Czyz M. WNT signaling in melanoma. Int J Mol Sci (2020) 21(14):4852. doi: 10.3390/ijms21144852
237. Bothun AM, Woods DC. Dynamics of WNT signaling components in the human ovary from development to adulthood. Histochem Cell Biol (2019) 151(2):115–23. doi: 10.1007/s00418-018-1729-y
238. Wu XQ, Wang YQ, Xu SM, Liu JF, Bi XY, Wang ZQ, et al. The WNT/β-catenin signaling pathway may be involved in granulosa cell apoptosis from patients with PCOS in North China. J Gynecol Obstet Hum Reprod (2017) 46(1):93–9. doi: 10.1016/j.jgyn.2015.08.013
239. Bicer M, Alarslan P, Guler A, Demir I, Aslanipour B, Calan M. Elevated circulating levels of secreted frizzled-related protein 4 in relation to insulin resistance and androgens in women with polycystic ovary syndrome. J Endocrinol Invest (2020) 43(3):305–13. doi: 10.1007/s40618-019-01108-4
240. Luan YY, Zhang L, Peng YQ, Li YY, Liu RX, Yin CH. Immune regulation in polycystic ovary syndrome. Clin Chim Acta (2022) 531:265–72. doi: 10.1016/j.cca.2022.04.234
241. Chermuła B, Kranc W, Celichowski P, Stelmach B, Piotrowska-Kempisty H, Mozdziak P, et al. Cellular processes in human ovarian follicles are regulated by expression profile of new gene markers-clinical approach. J Clin Med (2021) 11(1):73. doi: 10.3390/jcm11010073
242. Yuanyuan Z, Zeqin W, Xiaojie S, Liping L, Yun X, Jieqiong Z. Proliferation of ovarian granulosa cells in polycystic ovarian syndrome is regulated by microRNA-24 by targeting wingless-type family member 2B (WNT2B). Med Sci Monit (2019) 25:4553–9. doi: 10.12659/MSM.915320
243. Sanchez AM, Viganò P, Quattrone F, Pagliardini L, Papaleo E, Candiani M, et al. The WNT/β-catenin signaling pathway and expression of survival promoting genes in luteinized granulosa cells: endometriosis as a paradigm for a dysregulated apoptosis pathway. Fertil Steril (2014) 101(6):1688–96. doi: 10.1016/j.fertnstert.2014.02.040
244. Hernandez Gifford JA. The role of WNT signaling in adult ovarian folliculogenesis. Reproduction (2015) 150(4):R137–148. doi: 10.1530/REP-14-0685
245. Qiao GY, Dong BW, Zhu CJ, Yan CY, Chen BL. Deregulation of WNT2/FZD3/β-catenin pathway compromises the estrogen synthesis in cumulus cells from patients with polycystic ovary syndrome. Biochem Biophys Res Commun (2017) 493(1):847–54. doi: 10.1016/j.bbrc.2017.07.057
246. Gómez-Orte E, Sáenz-Narciso B, Moreno S, Cabello J. Multiple functions of the noncanonical Wnt pathway. Trends Genet (2013) 29(9):545–53. doi: 10.1016/j.tig.2013.06.003
247. Xiao Q, Chen Z, Jin X, Mao R, Chen Z. The many postures of noncanonical Wnt signaling in development and diseases. BioMed Pharmacother (2017) 93:359–69. doi: 10.1016/j.biopha.2017.06.061
248. Zhao Y, Zhang C, Huang Y, Yu Y, Li R, Li M, et al. Up-regulated expression of WNT5a increases inflammation and oxidative stress via PI3K/AKT/NF-κB signaling in the granulosa cells of PCOS patients. J Clin Endocrinol Metab (2015) 100(1):201–11. doi: 10.1210/jc.2014-2419
249. Niu Q, Shi J, Gao Q, Fu J. WNT5A enhances LH-mediated expression of HAS2 in granulosa cells. Reprod Sci (2022) 29(5):1618–29. doi: 10.1007/s43032-021-00736-7
250. van Dijk EM, Menzen MH, Spanjer AIR, Middag LDC, Brandsma CAA, Gosens R. Noncanonical WNT-5B signaling induces inflammatory responses in human lung fibroblasts. Am J Physiol Lung Cell Mol Physiol (2016) 310(11):L1166–1176. doi: 10.1152/ajplung.00226.2015
251. Abedini A, Zamberlam G, Lapointe E, Tourigny C, Boyer A, Paquet M, et al. WNT5a is required for normal ovarian follicle development and antagonizes gonadotropin responsiveness in granulosa cells by suppressing canonical WNT signaling. FASEB J (2016) 30(4):1534–47. doi: 10.1096/fj.15-280313
252. Abedini A, Zamberlam G, Boerboom D, Price CA. Non-canonical WNT5A is a potential regulator of granulosa cell function in cattle. Mol Cell Endocrinol (2015) 403:39–45. doi: 10.1016/j.mce.2015.01.017
253. Landry T, Shookster D, Huang H. Circulating α-klotho regulates metabolism via distinct central and peripheral mechanisms. Metabolism (2021) 121:154819. doi: 10.1016/j.metabol.2021.154819
254. Bednarska S, Fryczak J, Siejka A. Serum β-Klotho concentrations are increased in women with polycystic ovary syndrome. Cytokine (2020) 134:155188. doi: 10.1016/j.cyto.2020.155188
255. Mao Z, Fan L, Yu Q, Luo S, Wu X, Tang J, et al. Abnormality of klotho signaling is involved in polycystic ovary syndrome. Reprod Sci (2018) 25(3):372–83. doi: 10.1177/1933719117715129
256. Zeng X, Zhong Q, Li M, Liu Y, Long S, Xie Y, et al. Androgen increases klotho expression via the androgen receptor-mediated pathway to induce GCs apoptosis. J Ovarian Res (2023) 16(1):10. doi: 10.1186/s13048-022-01087-w
257. Xie T, Ye W, Liu J, Zhou L, Song Y. The emerging key role of klotho in the hypothalamus-pituitary-ovarian axis. Reprod Sci (2021) 28(2):322–31. doi: 10.1007/s43032-020-00277-5
258. Zhang Y, Ran Y, Kong L, Geng L, Huang H, Zhang H, et al. Decreased SFRP5 correlated with excessive metabolic inflammation in polycystic ovary syndrome could be reversed by metformin: implication of its role in dysregulated metabolism. J Ovarian Res (2021) 14(1):97. doi: 10.1186/s13048-021-00847-4
259. Zhang Y, Zhou H, Ding C. The ameliorative effect of CangFu Daotan Decoction on polycystic ovary syndrome of rodent model is associated with m6A methylation and Wnt/β-catenin pathway. Gynecol Endocrinol (2023) 39(1):2181637. doi: 10.1080/09513590.2023.2181637
260. Zhang Y, Wang X. Targeting the Wnt/β-catenin signaling pathway in cancer. J Hematol Oncol (2020) 13(1):165. doi: 10.1186/s13045-020-00990-3
261. Koike H, Harada M, Kusamoto A, Kunitomi C, Xu Z, Tanaka T, et al. Notch signaling induced by endoplasmic reticulum stress regulates cumulus-oocyte complex expansion in polycystic ovary syndrome. Biomolecules (2022) 12(8):1037. doi: 10.3390/biom12081037
262. Di Pietro M, Pascuali N, Parborell F, Abramovich D. Ovarian angiogenesis in polycystic ovary syndrome. Reproduction (2018) 155(5):R199–209. doi: 10.1530/REP-17-0597
263. Tal R, Seifer DB, Arici A. The emerging role of angiogenic factor dysregulation in the pathogenesis of polycystic ovarian syndrome. Semin Reprod Med (2015) 33(3):195–207. doi: 10.1055/s-0035-1552582
264. Xie Q, Cheng Z, Chen X, Lobe CG, Liu J. The role of Notch signalling in ovarian angiogenesis. J Ovarian Res (2017) 10(1):13. doi: 10.1186/s13048-017-0308-5
265. Vanorny DA, Mayo KE. The role of Notch signaling in the mammalian ovary. Reproduction (2017) 153(6):R187–204. doi: 10.1530/REP-16-0689
266. Patil K, Joseph S, Shah J, Mukherjee S. An integrated in silico analysis highlighted angiogenesis regulating miRNA-mRNA network in PCOS pathophysiology. J Assist Reprod Genet (2022) 39(2):427–40. doi: 10.1007/s10815-022-02396-1
267. Xu B, Zhang YW, Tong XH, Liu YS. Characterization of microRNA profile in human cumulus granulosa cells: Identification of microRNAs that regulate Notch signaling and are associated with PCOS. Mol Cell Endocrinol (2015) 404:26–36. doi: 10.1016/j.mce.2015.01.030
268. Chen L, Kong C. LINC00173 regulates polycystic ovarian syndrome progression by promoting apoptosis and repressing proliferation in ovarian granulosa cells via the microRNA-124-3p (miR-124-3p)/jagged canonical Notch ligand 1 (JAG1) pathway. Bioengineered (2022) 13(4):10373–85. doi: 10.1080/21655979.2022.2053797
269. Tamaddon M, Azimzadeh M, Tavangar SM. microRNAs and long non-coding RNAs as biomarkers for polycystic ovary syndrome. J Cell Mol Med (2022) 26(3):654–70. doi: 10.1111/jcmm.17139
270. George RM, Hahn KL, Rawls A, Viger RS, Wilson-Rawls J. Notch signaling represses GATA4-induced expression of genes involved in steroid biosynthesis. Reproduction (2015) 150(4):383–94. doi: 10.1530/REP-15-0226
271. Guo S, Quan S, Zou S. Roles of the notch signaling pathway in ovarian functioning. Reprod Sci (2021) 28(10):2770–8. doi: 10.1007/s43032-021-00610-6
272. Bergeron F, Nadeau G, Viger RS. GATA4 knockdown in MA-10 Leydig cells identifies multiple target genes in the steroidogenic pathway. Reproduction (2015) 149(3):245–57. doi: 10.1530/REP-14-0369
273. Ho DM, Artavanis-Tsakonas S, Louvi A. The Notch pathway in CNS homeostasis and neurodegeneration. Wiley Interdiscip Rev Dev Biol (2020) 9(1):e358. doi: 10.1002/wdev.358
274. Saad MA, Eltarzy MA, Abdel Salam RM, Ahmed MAE. Liraglutide mends cognitive impairment by averting Notch signaling pathway overexpression in a rat model of polycystic ovary syndrome. Life Sci (2021) 265:118731. doi: 10.1016/j.lfs.2020.118731
275. Tzavlaki K, Moustakas A. TGF-β Signaling. Biomolecules (2020) 10(3):487. doi: 10.3390/biom10030487
276. Raja-Khan N, Urbanek M, Rodgers RJ, Legro RS. The role of TGF-β in polycystic ovary syndrome. Reprod Sci (2014) 21(1):20–31. doi: 10.1177/1933719113485294
277. Derynck R, Budi EH. Specificity, versatility, and control of TGF-β family signaling. Sci Signal (2019) 12(570):eaav5183. doi: 10.1126/scisignal.aav5183
278. Miyazawa K, Miyazono K. Regulation of TGF-β Family signaling by inhibitory smads. Cold Spring Harb Perspect Biol (2017) 9(3):a022095. doi: 10.1101/cshperspect.a022095
279. Shen H, Wang Y. Activation of TGF-β1/Smad3 signaling pathway inhibits the development of ovarian follicle in polycystic ovary syndrome by promoting apoptosis of granulosa cells. J Cell Physiol (2019) 234(7):11976–85. doi: 10.1002/jcp.27854
280. Bao D, Li M, Zhou D, Zhuang C, Ge Z, Wei Q, et al. miR-130b-3p is high-expressed in polycystic ovarian syndrome and promotes granulosa cell proliferation by targeting SMAD4. J Steroid Biochem Mol Biol (2021) 209:105844. doi: 10.1016/j.jsbmb.2021.105844
281. Cheng JC, Fang L, Yan Y, He J, Guo Y, Jia Q, et al. TGF-β1 stimulates aromatase expression and estradiol production through SMAD2 and ERK1/2 signaling pathways in human granulosa-lutein cells. J Cell Physiol (2021) 236(9):6619–29. doi: 10.1002/jcp.30305
282. Zhou F, Shi LB, Zhang SY. Ovarian fibrosis: A phenomenon of concern. Chin Med J (Engl) (2017) 130(3):365–71. doi: 10.4103/0366-6999.198931
283. Wang D, Wang W, Liang Q, He X, Xia Y, Shen S, et al. DHEA-induced ovarian hyperfibrosis is mediated by TGF-β signaling pathway. J Ovarian Res (2018) 11(1):6. doi: 10.1186/s13048-017-0375-7
284. Takahashi N, Harada M, Hirota Y, Nose E, Azhary JM, Koike H, et al. Activation of endoplasmic reticulum stress in granulosa cells from patients with polycystic ovary syndrome contributes to ovarian fibrosis. Sci Rep (2017) 7(1):10824. doi: 10.1038/s41598-017-11252-7
285. Karakaya C, Çil AP, Bilguvar K, Çakir T, Karalok MH, Karabacak RO, et al. Further delineation of familial polycystic ovary syndrome (PCOS) via whole-exome sequencing: PCOS-related rare FBN3 and FN1 gene variants are identified. J Obstet Gynaecol Res (2022) 48(5):1202–11. doi: 10.1111/jog.15187
286. Prabhu Y D, Valsala Gopalakrishnan A. Can polyunsaturated fatty acids regulate Polycystic Ovary Syndrome via TGF-β signalling? Life Sci (2021) 276:119416. doi: 10.1016/j.lfs.2021.119416
287. Teede H, Ng S, Hedger M, Moran L. Follistatin and activins in polycystic ovary syndrome: relationship to metabolic and hormonal markers. Metabolism (2013) 62(10):1394–400. doi: 10.1016/j.metabol.2013.05.003
288. Chang HM, Fang L, Cheng JC, Taylor EL, Sun YP, Leung PCK. Effects of growth differentiation factor 8 on steroidogenesis in human granulosa-lutein cells. Fertil Steril (2016) 105(2):520–8. doi: 10.1016/j.fertnstert.2015.10.034
289. Chen MJ, Han DS, Yang JH, Yang YS, Ho HN, Yang WS. Myostatin and its association with abdominal obesity, androgen and follistatin levels in women with polycystic ovary syndrome. Hum Reprod (2012) 27(8):2476–83. doi: 10.1093/humrep/des209
290. Wang D, Wang W, Liang Q, He X, Xia Y, Shen S, et al. High GDF-8 in follicular fluid is associated with a low pregnancy rate in IVF patients with PCOS. Reproduction (2020) 160(1):11–9. doi: 10.1530/REP-20-0077
291. Bai L, Wang W, Xiang Y, Wang S, Wan S, Zhu Y. Aberrant elevation of GDF8 impairs granulosa cell glucose metabolism via upregulating SERPINE1 expression in patients with PCOS. Mol Ther Nucleic Acids (2021) 23:294–309. doi: 10.1016/j.omtn.2020.11.005
292. Altalhi R, Pechlivani N, Ajjan RA. PAI-1 in diabetes: pathophysiology and role as a therapeutic target. Int J Mol Sci (2021) 22(6):3170. doi: 10.3390/ijms22063170
293. Jia Q, Liu B, Dang X, Guo Y, Han X, Song T, et al. Growth differentiation factor-11 downregulates steroidogenic acute regulatory protein expression through ALK5-mediated SMAD3 signaling pathway in human granulosa-lutein cells. Reprod Biol Endocrinol (2022) 20(1):34. doi: 10.1186/s12958-022-00912-7
294. Jerobin J, Ramanjaneya M, Bettahi I, Parammal R, Siveen KS, Alkasem M, et al. Regulation of circulating CTRP-2/CTRP-9 and GDF-8/GDF-15 by intralipids and insulin in healthy control and polycystic ovary syndrome women following chronic exercise training. Lipids Health Dis (2021) 20(1):34. doi: 10.1186/s12944-021-01463-3
295. Zheng X, Zheng Y, Qin D, Yao Y, Zhang X, Zhao Y, et al. Regulatory role and potential importance of GDF-8 in ovarian reproductive activity. Front Endocrinol (Lausanne) (2022) 13:878069. doi: 10.3389/fendo.2022.878069
296. Ahmed ASF, Sharkawi SS, AbdelHameed SS, Bayoumi AM, Moussa RS, Alhakamy NA, et al. Ketogenic diet restores hormonal, apoptotic/proliferative balance and enhances the effect of metformin on a letrozole-induced polycystic ovary model in rats. Life Sci (2023) 313:121285. doi: 10.1016/j.lfs.2022.121285
297. Vetuschi A, Pompili S, Gaudio E, Latella G, Sferra R. PPAR-γ with its anti-inflammatory and anti-fibrotic action could be an effective therapeutic target in IBD. Eur Rev Med Pharmacol Sci (2018) 22(24):8839–48. doi: 10.26355/eurrev_201812_16652
298. Wang F, Zhang ZF, He YR, Wu HY, Wei SS. Effects of dipeptidyl peptidase-4 inhibitors on transforming growth factor-β1 signal transduction pathways in the ovarian fibrosis of polycystic ovary syndrome rats. J Obstet Gynaecol Res (2019) 45(3):600–8. doi: 10.1111/jog.13847
299. Zhou Y, Lan H, Dong Z, Cao W, Zeng Z, Song JL. Dietary proanthocyanidins alleviated ovarian fibrosis in letrozole-induced polycystic ovary syndrome in rats. J Food Biochem (2021) 45(5):e13723. doi: 10.1111/jfbc.13723
300. Zhang L, Wei W. Anti-inflammatory and immunoregulatory effects of paeoniflorin and total glucosides of paeony. Pharmacol Ther (2020) 207:107452. doi: 10.1016/j.pharmthera.2019.107452
301. Zhou J, Tan Y, Wang X, Zhu M. Paeoniflorin attenuates DHEA-induced polycystic ovary syndrome via inactivation of TGF-β1/Smads signaling pathway in vivo. Aging (Albany NY) (2021) 13(5):7084–95. doi: 10.18632/aging.202564
302. Zhou Y, Lan H, Dong Z, Li W, Qian B, Zeng Z, et al. Rhamnocitrin attenuates ovarian fibrosis in rats with letrozole-induced experimental polycystic ovary syndrome. Oxid Med Cell Longev (2022) 2022:5558599. doi: 10.1155/2022/5558599
303. Zhou YY, He CH, Lan H, Dong ZW, Wu YQ, Song JL. Rhamnocitrin decreases fibrosis of ovarian granulosa cells by regulating the activation of the PPARγ/NF-κB/TGF-β1/Smad2/3 signaling pathway mediated by Wisp2. Ann Transl Med (2022) 10(14):789. doi: 10.21037/atm-22-2496
304. Chang CH, Pauklin S. ROS and TGFβ: from pancreatic tumour growth to metastasis. J Exp Clin Cancer Res (2021) 40(1):152. doi: 10.1186/s13046-021-01960-4
305. Wang D, Wang T, Wang R, Zhang X, Wang L, Xiang Z, et al. Suppression of p66Shc prevents hyperandrogenism-induced ovarian oxidative stress and fibrosis. J Transl Med (2020) 18(1):84. doi: 10.1186/s12967-020-02249-4
306. Liang Y, Xu ML, Gao X, Wang Y, Zhang LN, Li YC, et al. Resveratrol improves ovarian state by inhibiting apoptosis of granulosa cells. Gynecol Endocrinol (2023) 39(1):2181652. doi: 10.1080/09513590.2023.2181652
307. Bangs F, Anderson KV. Primary cilia and mammalian hedgehog signaling. Cold Spring Harb Perspect Biol (2017) 9(5):a028175. doi: 10.1101/cshperspect.a028175
308. Pepling ME. Hedgehog signaling in follicle development. Biol Reprod (2012) 86(6):173. doi: 10.1095/biolreprod.112.100859
309. Liu C, Peng J, Matzuk MM, Yao HHC. Lineage specification of ovarian theca cells requires multicellular interactions via oocyte and granulosa cells. Nat Commun (2015) 6:6934. doi: 10.1038/ncomms7934
310. Makrinou E, Drong AW, Christopoulos G, Lerner A, Chapa-Chorda I, Karaderi T, et al. Genome-wide methylation profiling in granulosa lutein cells of women with polycystic ovary syndrome (PCOS). Mol Cell Endocrinol (2020) 500:110611. doi: 10.1016/j.mce.2019.110611
311. Liu T, Qin QY, Qu JX, Wang HY, Yan J. Where are the theca cells from: the mechanism of theca cells derivation and differentiation. Chin Med J (Engl) (2020) 133(14):1711–8. doi: 10.1097/CM9.0000000000000850
312. Wijgerde M, Ooms M, Hoogerbrugge JW, Grootegoed JA. Hedgehog signaling in mouse ovary: Indian hedgehog and desert hedgehog from granulosa cells induce target gene expression in developing theca cells. Endocrinology (2005) 146(8):3558–66. doi: 10.1210/en.2005-0311
313. Liu C, Rodriguez KF, Brown PR, Yao HHC. Reproductive, physiological, and molecular outcomes in female mice deficient in dhh and ihh. Endocrinology (2018) 159(7):2563–75. doi: 10.1210/en.2018-00095
314. Li Y, Xiong G, Tan J, Wang S, Wu Q, Wan L, et al. Aberrant activation of the Hedgehog signaling pathway in granulosa cells from patients with polycystic ovary syndrome. Bioengineered (2021) 12(2):12123–34. doi: 10.1080/21655979.2021.2003943
315. Finco I, LaPensee CR, Krill KT, Hammer GD. Hedgehog signaling and steroidogenesis. Annu Rev Physiol (2015) 77:105–29. doi: 10.1146/annurev-physiol-061214-111754
316. Ren Y, Cowan RG, Harman RM, Quirk SM. Dominant activation of the hedgehog signaling pathway in the ovary alters theca development and prevents ovulation. Mol Endocrinol (2009) 23(5):711–23. doi: 10.1210/me.2008-0391
317. Ren Y, Cowan RG, Migone FF, Quirk SM. Overactivation of hedgehog signaling alters development of the ovarian vasculature in mice. Biol Reprod (2012) 86(6):174. doi: 10.1095/biolreprod.112.099176
318. Xu A, Fan Y, Liu S, Sheng L, Sun Y, Yang H. GIMAP7 induces oxidative stress and apoptosis of ovarian granulosa cells in polycystic ovary syndrome by inhibiting sonic hedgehog signalling pathway. J Ovarian Res (2022) 15(1):141. doi: 10.1186/s13048-022-01092-z
Keywords: autophagy, hyperandrogenism, insulin resistance, polycystic ovary syndrome, signaling pathways
Citation: Wang K and Li Y (2023) Signaling pathways and targeted therapeutic strategies for polycystic ovary syndrome. Front. Endocrinol. 14:1191759. doi: 10.3389/fendo.2023.1191759
Received: 22 March 2023; Accepted: 18 September 2023;
Published: 19 October 2023.
Edited by:
Eytan R. Barnea, BioIncept, LLC, United StatesReviewed by:
Nikita Saraswat, Dr. DY Patil College of Pharmacy, IndiaBan J. M., University of Baghdad, Iraq
Copyright © 2023 Wang and Li. This is an open-access article distributed under the terms of the Creative Commons Attribution License (CC BY). The use, distribution or reproduction in other forums is permitted, provided the original author(s) and the copyright owner(s) are credited and that the original publication in this journal is cited, in accordance with accepted academic practice. No use, distribution or reproduction is permitted which does not comply with these terms.
*Correspondence: Yanhua Li, liyanhua330@163.com